Amino Acid, Essential Vitamin and Mineral Burn off in Post Exertional Malaise
- Graham Exelby
- Jul 7, 2024
- 62 min read
Updated: Feb 9
A Preliminary Study -September 2024, currently being peer-reviewed
Dr Graham Exelby
Brainstem hypoperfusion, coat hanger pain and PEM have similar pathogenesis centered around hypoperfusion, and these are found in that same group of medical conditions linked by fatigue and cognitive impairment- ME/CFS, POTS, Fibromyalgia Syndrome, Gulf War Syndrome and Long COVID. The brainstem, which consists of the midbrain, pons and medulla, has been implicated in ME/CIFS in many studies. This region regulates the respiratory, cardiovascular, gastrointestinal, and neurological processes, which can be affected by long-COVID, Fibromyalgia syndrome, POTS, migraine and ME/CFS.
Research into coat hanger pain has provided pathways demonstrating where hypoperfusion induces muscle depolarization, weakness, and mitochondrial dysfunction. ME/CFS patients have reduced reserves of Adenosine triphosphate (ATP) vital for mitochondrial energy production, and replenishment of ATP may take days. In post exertional malaise (PEM) the metabolic dysfunction induced by the hypoperfusion moves the body into catabolic metabolism with increased “burn off” of amino acids, essential minerals and vitamins. Assessing amino acids may provide a picture of the metabolic dysfunction that characterizes the fatigue.
The hypoxia is believed to impair the function of enzyme pyruvate dehydrogenase (PDH) in ME/CFS causing changes in the vital Krebs/ Citric acid cycle responsible for our mitochondrial energy source leading to inadequate adenosine triphosphate (ATP) generation by oxidative phosphorylation and excessive lactate generation on exertion.(1) That there is also an inflammatory component may be seen in patient studies where the thromboinflammatory pathway of COVID may be associated with brainstem hypoperfusion and cerebral hyperperfusion, and where central sleep apnoea secondary to the hypoperfusion may be modulated by corticosteroids, and noted by Hoel, Fluge et al (2) in one of the metabolic ME/CFS subtypes.
Research by Fluge et al (1) may to allow us to see which amino acids are being “burnt off” in the body’s quest to maintain ATP when the PDH pathway is impaired. Abnormalities in aspartic acid /glutamate levels may provide a link to the hypermetabolic changes seen in our brain SPECT scans, while abnormal ethanolamine may reflect dysfunction in the phospholipid pathway seen in COVID-19 research. Hoel, Fluge et al (2) described 3 emergent subtypes, but with a complexity far more than their findings, the preliminary findings in amino acid dysfunction in ME/CFS and post-exertional malaise (PEM) linked also to phospholipid dysfunction that fits with the POTS and Long COVID DNA findings by Dr Valerio Vittone.(3)
Summary of Proposed Pathophysiology of PEM
Clinic research into the amino acid dysfunction seen in PEM can be summarized, and discussed in depth in Targeting the Perfect Storm. (90)
Amino Acid Metabolism in PEM
Preliminary findings indicate persistent aspartate depletion post-exertion, aligning with the broader metabolic crisis hypothesis of PEM. Aspartate serves as a critical intermediary for:
TCA cycle anaplerosis, the metabolic process that replenishes the citric acid cycle’s intermediates which are essential for energy production
The malate-aspartate shuttle (MAS), critical for mitochondrial NADH transfer.
Nitrogen clearance in the urea cycle.
Dysregulated amino acid metabolism contributes to secondary glutamate imbalances. This dysfunction manifests as:
Impaired glutamate recycling and compartmentalization.
Neuroexcitation and excitotoxicity despite normal systemic glutamate levels.
Prolonged energy recovery due to slow replenishment of aspartate reserves.
Mitochondrial Dysfunction
PEM is characterized by reduced reserves of ATP, with prolonged recovery times due to impaired mitochondrial function. Evidence suggests a reliance on non-pyruvate-dependent TCA cycle intermediates, such as:
Branched-chain amino acids (e.g., leucine, isoleucine, valine).
Amino acids entering the TCA cycle as acetyl-CoA (e.g., lysine, phenylalanine, tryptophan).
Brainstem Hypoperfusion
Brainstem hypoperfusion has been implicated in autonomic dysfunction and PEM, as evidenced by SPECT imaging studies. Reduced perfusion impacts on global and regional cerebral blood flow regulation, as well as mitochondrial energy production within brainstem neurons
Findings from Wirth et al (8) highlight a 24.5% reduction in brainstem blood flow upon positional changes in ME/CFS, correlating with impaired cognitive and autonomic regulation.
Assays of neuroexcitatory amino acids glutamate, aspartic acid and histidine may provide explanations for the characteristic sensitization found in these conditions.
Glutamate metabolism is complex and interacts with many other biochemical pathways. The specific amino acid profiles found in these studies may provide clues about the nature of the dysfunction, potentially guiding treatment approaches.
The preliminary studies have been hampered by inadequate numbers of “control” testing of patients who have recovered from their POTS and Long COVID. Those tested appear to demonstrate similar patterns of amino acid dysfunction as those who are symptomatic, implying an underlying metabolic disorder that may be associated with their individual DNA patterns impacted by the various infective and other “activators” and “drivers” found in the individual’s POTS, Long COVID and Fibromyalgia investigation.
Simply supplementing the low amino acids may be fraught with potential risks as it does not consider the very complex dysfunctional metabolisms that are occurring, and which require a more determined assessment. I believe these preliminary studies will foster a more detailed research that affects ME/CFS, POTS, Fibromyalgia as well as Long COVID and lay the path for improved management protocols.
Chronic Fatigue and Amino Acid Dysfunction-combining research from Fluge et al (1) and Hoel, Fluge et al (2)
Cort Johnson (4) in his 2021 paper described the findings of a large Norwegian study (2) by Hoel, Fluge et al, which looked at the metabolic changes that occur in Chronic Fatigue Syndrome (ME/CFS). They found 67 metabolites affected in CFS suggesting a core energy dysfunction, with widespread changes in lipids and amino acids. This was described as the cells trying to access energy any way they can, turning to metabolism of fatty acids and amino acids instead of carbohydrates.
Brand et al (5) described the classic role of mitochondria as oxidative phosphorylation, which generates adenosine triphosphate (ATP) by utilizing the energy released during the oxidation of the food we eat. ATP is used in turn as the primary energy source for most biochemical and physiological processes, such as growth, movement and homeostasis. We turn over approximately our own body weight in ATP each day, and almost all of this is generated by mitochondria, primarily within muscle, brain, liver, heart and gastrointestinal tract.
The pre-eminent role of eating is to provide the fuel for mitochondria, and breathing is to provide the oxygen and to remove the carbon dioxide produced during oxidative phosphorylation by mitochondria. Similarly, a major role of the cardiovascular system is to deliver the substrates (glucose, fatty acids, oxygen) and remove the products (carbon dioxide) of mitochondrial activity. While the functions of mitochondria include oxidative phosphorylation to produce cellular ATP, but they also have important roles in ion homeostasis, several metabolic pathways, apoptosis and programmed cell death, and in ROS production and consumption. All of these functions may be significant in ageing and/or disease. Damage may cause mitochondria to accumulate dysfunctional components.(5)
Figure 1. Proposed mechanism of ME/CFS linked to amino acid catabolism.
Category I: amino acids are converted to pyruvate, and therefore depend on PDH to be further oxidized. These are alanine (Ala), cysteine (Cys), glycine (Gly), serine (Ser), and threonine (Thr).
Category II: amino acids that enter the oxidation pathway as acetyl-CoA, which directly and independently of PDH fuels the TCA cycle for degradation to CO2. These are isoleucine (Ile), leucine
(Leu), lysine (Lys), phenylalanine (Phe), tryptophan (Trp), and tyrosine (Tyr).
Category III consists of amino acids that are converted to TCA cycle intermediates, thereby replenishing and supporting the metabolic capacity of the cycle- histidine (His), and proline (Pro)
The asterisks indicate the amino acids significantly reduced in ME/CFS patients.
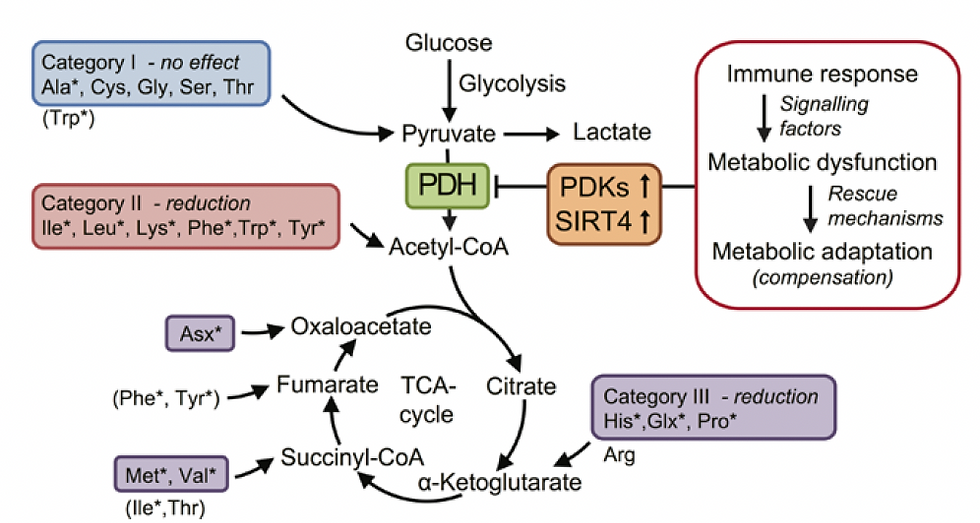
Source: Fluge et al., Metabolic profiling indicates impaired pyruvate dehydrogenase function in myalgic encephalopathy/chronic fatigue syndrome. JCI Insight. 2016 Dec 22;1(21):e89376. doi: 10.1172/jci.insight.89376. PMID: 28018972; PMCID: PMC5161229.(1)
Hoel, Fluge et al (2) found 3 subsets of patients in ME/CFS with metabolic patterns similar to those found in starvation and after intensive exercise. :
High degree of fatty acid and amino acid breakdown with high levels of ketone derivatives suggesting a ketogenic slant in 40%. Reduced levels of amino acid metabolites and low tryptophan was found. With little evidence of mitochondrial dysfunction this pattern was similar to starvation and after high-energy exercise.
Increased fatty acid breakdown but characterized by increased amino acid breakdown and evidence of mitochondrial dysfunction in 45%. There were increased pyruvate levels suggesting this wasn’t being broken down properly and that the mitochondria may not be getting the acetyl-CoA and NADH they needed. Increased tryptophan but lack of tryptophan derivatives suggested it wasn’t being broken down correctly. This group with the most severe symptoms, had metabolomic profiles similar to those of inflammatory diseases.
The 3rd group, 15%, had intermediate profiles.
Hoel, Fluge et al (2) suggested that elevated energy strain may result from exertion-triggered tissue hypoxia and lead to systemic metabolic adaptation and compensation. Through various mechanisms, such metabolic dysfunction represents a likely mediator of key symptoms in ME/CFS.
Pyruvate Dehydrogenase and Acetyl-Coenzyme A ( Acetyl-CoA)
The impairment of pyruvate dehydrogenase (PDH) in ME/CFS is believed to be linked to the increased expression of pyruvate dehydrogenase kinases, specifically PDK1, PDK2, PDK4 sirtuin 4; and PPARδ in peripheral blood mononuclear cells. These inhibit PDH activity- the aberrant increase in PDK expression might be influenced by the activity of PPAR transcription factors, leading to impaired PDH function in ME/CFS patients.(1) This impairment in PDH results in obstructed pyruvate catabolism, forcing the body to rely more on acetyl-CoA-producing amino acids as alternative substrates for aerobic metabolism via the TCA cycle.
Anaerobic threshold and recovery time after exercise depend on rates of lactate production and clearance. Lactate is made from pyruvate, mostly arising from glucose catabolism via the glycolytic pathway or from breakdown of certain amino acids. Under anaerobic conditions, when lack of oxygen prohibits mitochondrial respiration, pyruvate builds up in cytosol, which leads to increased production and cellular excretion of lactate. Mitochondrial dysfunction leads to both excessive lactate production and a deficient supply of ATP, and has been suggested to play a role in ME/CFS. PDH functions as a gateway in oxidative metabolism by coordinating the breakdown of 2- and 3-carbon energy substrates derived from carbohydrates, fats, and amino acids. A reduction in PDH enzymatic activity may lead to accumulation of pyruvate and thereby cause overproduction of lactate, even in the presence of adequate oxygen levels. (1)
Fluge et al (1) found the most prominent changes in ME/CFS patients were widespread decreases in sphingolipids, glycosphingolipids, and phospholipids. These findings were consistent with a lower ATP and GTP turnover and with decreased amounts of branched amino acid metabolic intermediates. However, there were also indications of reduced mitochondrial fatty acid oxidation only in female ME/CFS patients.(1) The phospholipid dysfunction has been demonstrated in clinic amino acid tests with low ethanolamine assays.
Sirtuin 4 (SIRT4) is a mitochondrial protein that inhibits mitochondrial glutamate dehydrogenase 1(GDH) activity, thereby downregulating insulin secretion in response to amino acids, as well as PDH activity. This regulation prevents overactivation of PDHC under nutrient surplus conditions, preserving mitochondrial homeostasis. In CFS/PEM, dysregulated SIRT4 activity may exacerbate PDH dysfunction, limit mitochondrial ATP production, and disrupt amino acid and energy metabolism.
A deacetylation of malonyl-CoA decarboxylase enzyme by SIRT4 inhibits fatty acid oxidation in muscle and liver cells, and has a suppressive effect on (PPAR-α) which downregulates fatty acid oxidation in liver cells.(1) SIRT4 thus plays a role in regulating cellular responses to oxidative stress and metabolic stress, including mitochondrial damage and energy deficits. The use of nicotinamide to modulate this dysfunctional pathway is discussed later.
Dysfunction in this pathway has been suggested to be part of the brain fog in Long COVID, ME/CFS and POTS. The positioning of pyruvate can be demonstrated below at the keystone of the Citric Acid Cycle. (Figure 1 and 2)
Several factors can lead to increased expression of PDK1, PDK2, and PDK4:
Hypoxia - Hypoxia-inducible factor (HIF), especially HIF-1α, activates the expression of PDK1 and PDK3 under low oxygen conditions (6)
Malignancy- Cancer cells often have mutations that activate HIF signaling, leading to high PDK expression (6)
Metabolic abnormalities- diabetes, insulin resistance, fasting and high-fat diets can upregulate PDK4 expression (6)(7)
Various transcription factors (6)
Hormones and nutritional factors- In starvation and diabetes, nutritional factors and hormones regulate PDK2 and PDK4 expression (6)
Inflammation - Inflammatory conditions like diabetic nephropathy, sepsis, and amyotrophic lateral sclerosis correlate with increased PDK4 expression (7)
Mitochondrial dysfunction - Impaired mitochondrial function can lead to compensatory upregulation of PDK (1)
Specific to ME/CFS- increased expression of PDK1, PDK2, and PDK4 is observed, possibly due to aberrant regulation mechanisms involving PPARs and SIRT4. (8)
Acetyl-CoA is a metabolic intermediate that is involved in many metabolic pathways. It is produced during the breakdown of glucose, fatty acids and amino acids, and is used in the synthesis of many other biomolecules including fatty acids, cholesterol and ketone bodies. It is a key molecule in the Kreb’s, or Citric acid cycle, a series of chemical reactions that occur in the mitochondria and is responsible for generating energy in the form of ATP. (Figure 2)
Figure 2: Citric Acid Cycle (or Kreb’s cycle or TCA cycle)
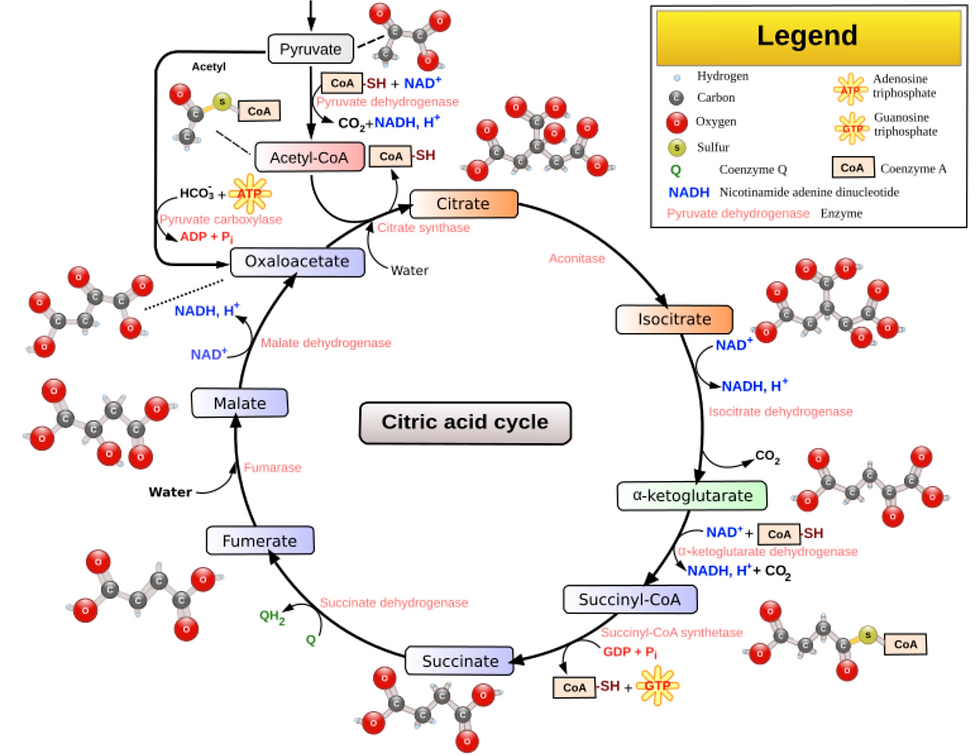
Source: Narayanese (original file), WikiUserPedia (SVG), CC BY-SA 3.0 <http://creativecommons.org/licenses/by-sa/3.0/>, via Wikimedia Commons
Brainstem Hypoperfusion
The brainstem, which consists of the midbrain, pons and medulla, has been implicated in ME/CIFS in many studies. It regulates the respiratory, cardiovascular, gastrointestinal, and neurological processes, which can be affected by long-COVID and similar disorders eg migraine and ME/CFS.
Griffith University showed in 2023 that the brainstem is larger in Long Covid and ME/CFS patients (9) and the brainstem demonstrates an imbalance of neurochemicals in this same group in 2024. (10) These changes correlate with the SPECT scan findings in ME/CFS, POTS and Long COVID found in our studies.
The brainstem hypoperfusion also seen in SPECT scans from our clinic findings, is believed to be part of the same process of hypoperfusion and mitochondrial dysfunction that underpins the coat hanger pain of FMS and POTS.
In severe ME/CFS, Wirth et al (8) noted the reduction in blood flow in the brainstem from lying to sitting was 24.5%. They described the reduced blood flow to the brainstem causing neurological symptoms including impaired cognitive function or “brain fog.” This hypoperfusion can impact both the global and local regulation of blood flow in the brain. COVID-19 seriously affects the endothelium and there is evidence of chronic endothelial dysfunction in the post-Covid-syndrome similar to that in ME/CFS.(11)
They also reported an increase in intracranial pressure observed in ME/CFS patients, and later proposed by Hulens (12) and Bragee (13) also has been frequently observed compounding problems. The combination of brain perfusion abnormality and intracranial CSF dysfunction has been frequently observed in our clinic, compounding problems and intricately linking the 2.
As a possible explanation for the orthostatic intolerance and the decrease in cerebral blood flow they proposed the presence of both a strong vasoconstrictor effect mediated by an elevated sympathetic tone and weakened vasodilator influences. Clinic findings confirm this is a significant component, but we have also found mechanical causes.- Brainstem Hypoperfusion, Coat Hanger pain and Post-Exertional Malaise in POTS and Long COVID. (14)
Wirth et al (8) also reported muscle mitochondrial dysfunction, as evidenced by higher levels of pyruvate and lower levels of ATP and phosphocreatine in muscles, suggesting an impairment in muscle energy metabolism, which is also observed in ME/CFS, indicating a likely overlap in the pathophysiological mechanisms of these conditions.
Long Covid research by Appleman et al, (15) has also shown post-exertional malaise (PEM), with associated fatigue, pain and local and systemic metabolic disturbances, severe exercise-induced myopathy and tissue infiltration of amyloid-containing deposits in skeletal muscles of patients with long COVID.
The impaired glymphatic function seen in Long COVID with its impairment of the paravascular space function also appears to have far-reaching effects on symptoms such as fatigue, brain fog and head pressure by CSF flow dysfunction.
Combining the research into the Glymphatic dysfunction, Intracranial Hypertension by Hulens (11), Bragee (12) and others with brainstem hypoperfusion and the catabolic metabolism of coat hanger pain and PEM provides a satisfactory hypothesis for much of the symptomatology in all of these.
The use of brain SPECT scans in POTS, Long COVID and ME/CIFS has demonstrated brainstem hypoperfusion which may assist us in determining whether we may be dealing with mitochondrial dysfunction eg after Infectious Mononucleosis, or a vascular/autonomic dysfunction with brainstem hypoperfusion with the sequalae from this, or more commonly, a combination of both. - Brainstem Hypoperfusion, Coat Hanger pain and Post-Exertional Malaise in POTS and Long COVID. (14)
Post-exertional malaise (PEM)
Post-exertional malaise (PEM) has been compared to the metabolic changes that a marathon runner may experience following their race. Coat hanger pain can contribute to PEM through hypoperfusion and muscle ischaemia. When muscles have inadequate oxygen then switch to anaerobic metabolism, with accumulation of lactic acid which causes the characteristic cramping and pain. The relationship between the two is shown with exercise, where in POTS even minor exertion increases pain, fatigue and cognitive difficulties which characterize PEM. (16) The interplay between reduced blood flow, lactic acid accumulation and autonomic dysfunction leads to a snowballing increase in symptoms and prolonged recovery times. ME/CFS patients have reduced reserves of Adenosine triphosphate (ATP) vital for mitochondrial energy production, and replenishment of ATP may take days.
PEM in ME/CFS involves complex metabolic changes including alterations in amino acid metabolism, with evidence of affects 24 hours after exercise and PEM activation. Non-essential amino acids, particularly those that can fuel the TCA cycle independently of pyruvate dehydrogenase (whose function is impaired in PEM) may become increasingly important for maintaining energy production during PEM episodes,(17(18(19) as shown by Fluge et al.(1)
Hypermetabolism appears to play a significant role in PEM characterized by increased excretion of urine metabolities indicating an abnormally high rate of metabolism. The hypermetabolism in PEM is also associated with:
the increased excretion of methylhistidine in urine during PEM indicates elevated muscle protein breakdown. This may also contribute to amino acid depletion as muscle is a significant reservoir of amino acids in the body. (1)
intestinal barrier breakdown,
acetate excretion,
glycolytic abnormalities with altered glucose: lactate ratios,
purine metabolism dysregulation with decreased hypoxanthine,
hypoacetylation affecting multiple cytoplasmic enzymes and DNA histone regulation affecting cellular function and gene expression
metabolite loss most likely from hypermetabolic state and hypoacetylation contributing to the prolonged recovery period in CFS patients after exercise.
Potential Causes of Fatigue in Long COVID
COVID has enabled researchers to look more closely at pathogenesis of many of the problems seen in both POTS and Long COVID. While these are being seen in COVID, basic principles apply in other causes. For many, knowledge of whether there is brainstem hypoperfusion (reduced blood flow), or abnormal amino acids/neurotransmitters can help decide a pathway to tackle the fatigue.
Table 1: Potential Causes of Fatigue in Long COVID- combining available and ongoing clinic research
Reactivation of EBV and similar viruses that may cause mitochondrial dysfunction in the first place
Mitochondrial dysfunction, as evidenced by higher levels of pyruvate and lower levels of ATP and phosphocreatine in muscles (8)
Brainstem hypoperfusion with mitochondrial dysfunction /oxidative stress (CFS and coat hanger pain with progressive muscle depolarization) and catabolic metabolism /amino acid dysfunction with impact on ATP production.(20)(21)
Astrocyte /glymphatic dysfunction (TLR2-driven or crosstalk with microglia/mast cells)
Neurotransmitter dysfunction -Dopamine/serotonin/glutamate pathways and amino acid dysfunction (20)
Intracranial pressure abnormality with HPA axis dysfunction (14)(22)(23)
Direct cardiac damage (eg pericarditis, myocarditis, reduced Ejection Fraction)
Pulmonary damage- embolic, inflammatory, malignancy
Renal dysfunction through multiple causes including renal vein thrombosis and nephrotic syndrome
Mitochondrial dysfunction /oxidative stress. There are mitochondrial and inflammatory deleterious gene variants that are critical to mitochondrial dysfunction and the exaggerated immune response caused by COVID (3)
Metabolic pathway abnormality/ DNA mutations /dietary triggers. These include PEMT mutations and dysfunction in phosphatidycholine and phosphatidylserine metabolism (3)(23)(24)
Other vital DNA mutations associated with dysfunction include APO E4 with its known increased cardiovascular, neurodegenerative and liver dysfunction potential.(3)
Liver disease, with reduced clearance of fibrin degradation products
Undiagnosed malignancy that can accompany Natural Killer Cell dysfunction.(21)(25)(26) It is though that NK cells are functioning at about 20% capacity in Long COVID, ME/CFS and GWS.(22) TLR4 activation can cause NFkB dysfunction and dysregulation of malignancy risk
Small fibre neuropathy
Autonomic instability eg POTS, dysautonomia, orthostatic hypotension
Impaired cardiac function
Very common (again IL-6 and TNFa)-accompanying exaggerated neuropathy, rheumatoid arthritis, reactive arthritis, PMR strongly suggestive of TLR4 and STAT3 mutations (3)
Cardiac preload failure (unexplained shortness of breath with postural change)- best described as failure of the priming of the “pump-” currently at research level.
Direct cardiac involvement (including fibrin dysfunction)
Locus coeruleus/ Nucleus Tractus Solitarius dysfunction
Dysfunctional baroreceptor signalling from the carotid baroreceptor and low pressure receptors in heart and great veins
Aberrant azygous anatomy/ sympathetic activation
Reduced blood flow back to heart with arms raised when venous TOS is present.
Figure 3: SPECT Scans showing brainstem hypoperfusion and hyperperfusion
These demonstrate the mixed hyperperfusion and brainstem hypoperfusion typical of POTS. Green represents normal perfusion. The blue areas reflect hypoperfusion, green normal, yellow, red then white increased metabolic activity/ hyperperfusion.
The hyperperfusion is thought to be from endotheiliitis associated with intracranial vascular pressure as there is increasing evidence from our studies of impaired venous return causing a “backup” of venous pressure. Stagnant blood may play a part with its known activation of inflammatory cytokines causing the endotheleiitis. The scan image itself probably reflects increased levels of excitotoxic neurotransmitters affecting the brain through penetration through the dysfunctional BBB from the dysfunctional venous return.
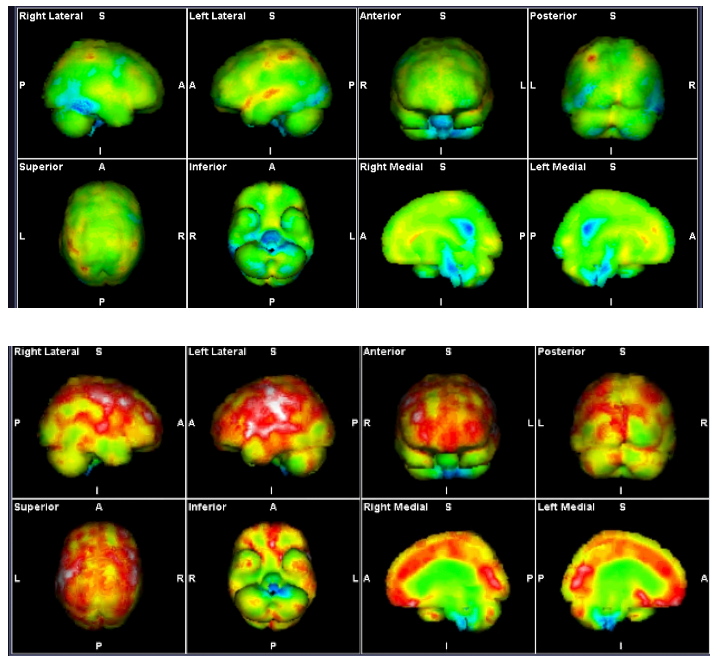
Source: Mermaid Molecular Imaging
Amino Acid Metabolism
There are 20 amino acids, organic compounds composed mainly of nitrogen, carbon, hydrogen, and oxygen. Animals have seven conditionally essential amino acids that can be synthesized and are usually not required in the diet. However, they are essential components of the diet for specific populations that cannot synthesize them in adequate amounts. There are four “nonessential” amino acids can be synthesized and, thus, are not required in diet. The remaining nine “essential” amino acids are obtained from diet.(27)
Amino acid metabolism refers to the biochemical pathways that produce, break down, and use amino acids. The body uses amino acids to make proteins, enzymes, hormones, and other important molecules. Protein synthesis and degradation are both essential for maintaining homeostasis in the body.(28)
Amino acids can also generate glucose, ATP, and fatty acids, and they are metabolic precursors for numerous biomolecules, including haeme groups, nucleotide bases, and signalling molecules (catecholamines, neurotransmitters). In addition, they are essential for epigenetic modifications.(27)
Amino acid oxidation is a process that helps the body release energy from protein molecules. This process occurs in the liver and muscles and results in the production of ketone bodies which can be used by cells to generate energy.(28)
Brief Guide to Amino Acids (27)
The citric acid cycle or Kreb’s cycle or TCA cycle (Figure 1) is a series of biochemical reactions to release the energy stored in nutrients through oxidation of acetyl-CoA derived from carbohydrates, fats and proteins. This energy released is in the form of ATP or Adenosine triphosphate, the “molecular unit of currency.” Glycolytic and TCA cycle intermediates generate the nonessential and conditionally essential amino acids.
Free amino acids produced from degradation of either cellular proteins or dietary proteins are deaminated to yield NH4+ and a carbon skeleton. NH4+ enters the urea cycle and the carbon skeleton can enter metabolic pathways to generate ATP, glucose, and fatty acids.
Glutamate acts as both a nitrogen donor and acceptor and is the central amino acid for the movement of nitrogen among amino acids.
Tyrosine is the precursor to generation of noradrenalin, adrenalin, dopamine, and melanins.
Methionine provides the methyl group for many DNA and histone methyltransferases to regulate epigenetics.
Cysteine, glutamate, and glycine generate the antioxidant glutathione.
Nitric oxide synthases use arginine to generate nitric oxide (NO).
Glycine and glutamate can serve as neurotransmitters.
Glutamate can generate another amino acid neurotransmitter, γ-aminobutyric acid (GABA), which does not participate in protein synthesis.
Tryptophan is a precursor for serotonin.
Serotonin is used to generate melatonin.
L dopa is converted to dopamine, by enzyme amino acid decarboxylase. This can occur in both dopaminergic and serotonergic neurons (29)
Tryptophan is converted to serotonin also involving enzyme amino acid decarboxylase.(29)
Histidine is converted to histamine, involving histidine decarboxylase
Phenylalanine is converted to phenylethylamine (PEA) by enzyme amino acid decarboxylase. This conversion is dependent on Vitamin B6 as a cofactor. This conversion can occur in various tissues including the brain, and is regulated by monoamine oxidase (MAO).(29)(30) Phenylethylamine (PEA) is a neurotransmitter and neuromodulator. It rapidly crosses the blood brain barrier but has a very short half-life in the brain, rapidly metabolized by monoamine oxidase M. (31)
It increases the release and inhibits the reuptake of dopamine and noradrenalin in the brain, leading to higher levels which can improve mood, focus and concentration.(28)
PEA activates trace amine-associated receptors further enhancing dopamine and serotonin release.(31)
By increasing dopamine PEA may improve working memory, executive function and attention, potentially helping ADHD
Increased dopamine and serotonin may improve mood and reduce anxiety and depression
L dopa and tryptophan are dietary derived. Amino acid decarboxylase is also B6 dependant.
Serotonin/ dopamine have an effect on glutamate through the DARPP-32 pathways. This is a complicated pathway involving multiple steps.
DARPP-32 is involved in the modulation of glutamatergic signalling by dopamine and serotonin, and is an integrator of various different enzymes and regulatory mechanisms.
The Essential Amino Acids (32)
Phenylalanine: Your body turns this amino acid into the neurotransmitters tyrosine, dopamine, epinephrine, and norepinephrine. It plays an integral role in the structure and function of proteins and enzymes and the production of other amino acids.
Valine: This is one of three branched-chain amino acids (BCAAs) on this list. helps stimulate muscle growth and regeneration and is involved in energy production.
Threonine: This is a principal part of structural proteins such as collagen and elastin, which are important components of your skin and connective tissue. It also plays a role in fat metabolism and immune function.
Tryptophan: Often associated with drowsiness, tryptophan is a precursor to serotonin, a neurotransmitter that regulates your appetite, sleep, and mood.
Methionine: This amino acid plays an important role in metabolism and detoxification. It’s also necessary for tissue growth and the absorption of zinc and selenium, minerals that are vital to your health.
Leucine: Like valine, leucine is a BCAA that is critical for protein synthesis and muscle repair. It also helps regulate blood sugar levels, stimulates wound healing, and produces growth hormones.
Isoleucine: The last of the three BCAAs, isoleucine is involved in muscle metabolism and is heavily concentrated in muscle tissue. It’s also important for immune function, haemoglobin production, and energy regulation.
Lysine: Lysine plays major roles in protein synthesis, calcium absorption, and the production of hormones and enzymes. It’s also important for energy production, immune function, and collagen and elastin production.
Histidine: Your body uses this amino acid to produce histamine, a neurotransmitter that is vital to immune response, digestion, sexual function, and sleep-wake cycles. It’s critical for maintaining the myelin sheath, a protective barrier that surrounds your nerve cells. (32) The metabolism of histidine, glutamine and glutamate are closely related, so imbalances could disrupt normal metabolic processes. Histidine can be degraded to glutamate via urocanic acid.(33) It has a role in carnosine synthesis which may play a part in PEM. (34)
Non-essential amino acids include (35)(36)
1. Alanine clears toxins released during muscle protein breakdown, aids in regulating blood glucose and cholesterol, serves as an energy source for muscles and the CNS and assist in lymphocyte production
2. Arginine is a precursor for nitric oxide, accelerated wound healing, renal detoxification, hormone homeostasis, supports immune system function
3. Asparagine is important for neuron development and CNS homeostasis, helps build connective tissue and aids generation of digestion with generation of secretions and mucus in the GI tract.
4. Aspartic acid is an excitatory neurotransmitter and is involved in synthesis of other amino acids including methionine, threonine, isoleucine and lysine. It plays a role in the citric acid cycle for energy production and the urea cycle. It acts as an excitatory neurotransmitter in the ventral spinal cord similar to glycine (which is inhibitory).(37) It has a role in the Urea Cycle where it combines with citrulline to form argininosuccinate, a critical step in nitrogen clearance via the urea cycle. Aspartate and glutamate can excite virtually every neuron in the CNS at low concentrations.(38) Aspartate is found in large quantities in neuroendocrine tissues eg hypothalamus. (39) Aspartate and glycine form an excitatory/inhibitory pain in the spinal cord, similar to glutamate/GABA in the brain.(37) Dysfunction in the body’s use and replenishment of aspartate, possibly with dysfunction in the Malate Aspartate Shuttle may explain the characteristic delays in timing of PEM. Targeting the Perfect Storm. (90)
5. Cysteine stimulates collagen production and is involved in the synthesis of glutathione, and plays a role in protein folding and stability
6. Glutamic acid (the anionic form is known as glutamate) is an α-amino acid that is used by almost all living beings in the biosynthesis of proteins. It is a non-essential nutrient for humans, meaning that the human body can synthesize enough for its use.-(discussed below)
7. Glutamine is the most abundant free amino acid in the body, providing nitrogen for synthesis of other amino acids, improves exercise endurance, supports lymphocyte/ immune function, is involved in glutathione synthesis, and is essential for intestinal cell metabolism
8. Glycine is involved in the production of DNA, phospholipids and collagen. It helps in the production of bile acids and supports glutathione synthesis. It acts as an inhibitory neurotransmitter in the CNS. Aspartate and glycine form an excitatory/inhibitory pain in the spinal cord, similar to glutamate/GABA in the brain.(37)
9. Proline is a major component of collagen, crucial for wound healing and tissue repair. It supports collagen function and structure and helps maintain skin elasticity.
10. Serine is involved in phospholipid formation, is a precursor of several amino acids including glycine and cysteine. It plays a role in DNA and RNA synthesis, and is important for proper functioning of the CNS.
11. Tyrosine is a precursor for neurotransmitters dopamine, noradrenalin and adrenalin. It is involved in melanin production, helps in synthesis of thyroid hormones, and supports functioning of the adrenal, thyroid and pituitary glands.
Preliminary Amino Acids Identified in “Burn Off”
Examination of amino acid profiles using plasma and urinary assay demonstrates a number of response patterns. Accuracy can depend on collection techniques and avoidance of amino acid supplements before testing. Elevated Taurine for example may be seen after consumption of energy drinks. Elevated glutamic acid and 1- and 3-methylhistidine have also been seen in the urine assays.
Low urinary taurine has been demonstrated in CFS (65). Taurine plays a crucial role in regulating cellular energy metabolism and mitochondrial function, so it’s deficiency may contribute to the fatigue and energy depletion characteristic of CFS and FMS. (79)(80) As taurine plays an important role in skeletal muscle function, including regulating membrane excitability,(66) low taurine could potentially contribute to muscle fatigue and weakness in CFS. It is important for cardiovascular health and blood pressure regulation. Low levels could exacerbate POTS symptoms by affecting blood volume and vascular tone. (79)
Taurine is not considered a true essential amino acid because healthy adults can synthesize it in their liver from other amino acids (methionine and cysteine). However, taurine becomes essential under certain conditions eg during periods of severe illness or stress, when the body's synthesis capacity may be impaired.(67)
Elevated glycine may point to altered neurotransmitter balance, as glycine is an inhibitory neurotransmitter (1), or potential issues with detoxification processes as glycine is involved in various detoxification processes. (1)
Abnormal asparagine has been found in one study to correlate with gastrointestinal symptoms. Elevated asparagine is thought by researchers may reflect a chronic state of energy deficiency or hypometabolism.(2)(61)
Plasma assays have shown low threonine, tyrosine, arginine, likely to reflect prolonged PEM activity and overall reduced levels.
Low levels of urinary ethanolamine, lysine, tyrosine, histidine, aspartic acid and have been observed in our preliminary studies, often seen collectively. Ethanolamine in particular may have more relevance in the phospholipid pathway dysfunction, and may be involved in the thromboinflammatory processes seen in Long COVID with its association with PEMT mutations.
The recent addition of GABA has shown low GABA levels. GABA, glutamate, aspartic acid and histidine levels are discussed below.
High plasma branched-chain amino acids (BCAAs) eg isoleucine, leucine and valine appear indicative of several metabolic disturbances. Possible scenarios include this may reflect mitochondrial dysfunction, a response to the inflammatory processes occurring as these amino acids can modulate immune function,, and they also compete with other amino acids eg tryptophan and tyrosine for transport across the blood-brain barrier potentially affecting serotonin and dopamine synthesis. BCAAs play a role in muscle protein synthesis and energy production. Elevated levels could reflect the deconditioning that occurs in POTS.
Lysine and Aspartic Acid -the most commonly affected
Aspartic acid is an excitatory neurotransmitter and is involved in synthesis of other amino acids including methionine, threonine, isoleucine and lysine, and it’s importance in neuroexcitatory changes in CFS is detailed below. It is a non-essential amino acid involved in various physiological processes, including hormone production and neurotransmission. While it plays a role in maintaining protein solubility and ionic bonding, its direct impact on collagen synthesis is not as pronounced as that of other amino acids like glycine, proline, and lysine. However, increased utilization of aspartate in the urea cycle may deplete its systemic levels, especially under conditions of nitrogen overload, mitochondrial dysfunction, or impaired urea synthesis. Increased lysine catabolism may draw upon aspartate, further depleting it and contributing to low urinary levels.
Low Lysine in preliminary studies is the most commonly effected amino acid. Lysine intake is crucial for maintaining healthy collagen function, which supports the structural integrity of skin, tendons, bones, and other connective tissues. Deficiencies can lead to weakened connective tissues, delayed wound healing, and other related health issues.(78) As well as being utilized in the Category 2 amino acid burn off as described by Fluge et al (1), clinically it appears to have significant effects on collagen metabolism. Nicotinamide (Vitamin B3) can influence lysine’s role in collagen synthesis, especially in the context of diabetes and wound healing. Symptoms may include muscle weakness, fatigue, or high serum triglycerides due to decreased fatty acid transport from low levels of carnitine and lysine.
Low lysine levels can significantly impact collagen function due to lysine's crucial role in collagen synthesis and stabilization with effects on:
Collagen cross-linking, which is critical for the structural integrity and tensile strength of connective tissues. A deficiency in lysine can lead to weaker collagen structures due to inadequate cross-linking. (81)
Collagen stability- The hydroxylation of lysine residues in collagen increases the stability and strength of the collagen fibres. Without sufficient lysine, this process is impaired, potentially leading to weaker connective tissues and compromised tissue integrity.(81) Lysine’s role in collagen synthesis is through hydroxylation which is facilitated by the conversion of NAD+ to NADH, which involves nicotinamide as a component of NAD+.(53)
As collagen is a major component of the extracellular matrix, this may lead to broader issues in tissue integrity. (51)(52)
Bone and Connective Tissue Health, as it is involved in the synthesis of tropocollagen, which assembles into mature collagen fibres essential for bone formation and remodelling, so a deficiency may impact on bone density and overall connective tissue health. (81)(82)
Wound healing- Lysine plays a role in wound healing by aiding in collagen synthesis. Low lysine levels can slow down wound repair processes due to reduced collagen production and deposition at wound sites.(81)
Nicotinamide (Vitamin B3) can influence lysine’s role in collagen synthesis, especially in the context of diabetes and wound healing. Symptoms may include muscle weakness, fatigue, or high serum triglycerides due to decreased fatty acid transport from low levels of carnitine and lysine. Nicotinamide riboside holds significant promise in CFS/PEM by modulating SIRT4 activity and improving mitochondrial and metabolic function. By restoring NAD⁺ levels, NR optimizes SIRT4-mediated regulation of PDH, glutamine metabolism, and stress responses, potentially alleviating energy deficits and systemic dysfunction.
Nicotinamide, as a precursor for NAD⁺, directly influences SIRT4 activity and mitochondrial metabolism. The interplay between nicotinamide, NAD⁺, and SIRT4 can influence the observed amino acid imbalances.
Clinic studies have found introducing liposomal nicotinamide riboside has positive effects on fatigue and amino acid patterns, including stabilizing of ethanolamine function. Liposomal delivery systems significantly enhance the bioavailability and clinical efficacy of supplements, including nicotinamide riboside (NR) for mitochondrial and metabolic dysfunction (as seen in CFS) and vitamin B3 (nicotinamide/niacin) for skin health and cancer prevention.
Amino acids changes links to sensitization.
Elevated levels of glutamic acid with low aspartic acid also seen and may reflect the dysfunctional glutamate seen in Functional Neurological Disorder,(FND) but these may be at least in part collection errors in glutamatic acid/glutamine. The assay for Glutamate / Glutamic acid is often not reliable as glutamine decomposes to glutamate quickly and this can produce elevated levels dependent on collection technique.
Elevated plasma histidine may be an important component of PEM and the excitotoxicity.
In the normal glutamic acid, low aspartic acid and histidine pattern, this suggests there may be a disruption in metabolism of amino acids without directly affecting glutamate levels. Aspartic acid and glutamic acid are both excitatory neurotransmitters, so a decrease in aspartic acid may be a compensatory mechanism to maintain overall excitatory balance.(55) This may lead to elevated glutamate levels and disruptions in the balance between excitatory (glutamate) and inhibitory (GABA) neurotransmission.
Low histidine could indicate altered histamine signalling, which interacts with the glutamatergic system.(56) Histidine may be retained or depleted systemically to meet increased physiological demands, leading to lower urinary excretion. Chronic inflammation or mast cell activation (common in conditions like POTS and endometriosis) leads to increased histamine production from histidine. Chronic immune or inflammatory responses divert histidine toward histamine production, lowering free histidine levels in circulation.
Histidine is metabolized into glutamate via the intermediate formiminoglutamate (FIGLU). Disruptions in this pathway can affect histidine levels. FIGLU requires tetrahydrofolate (THF) for conversion to glutamate. Folate deficiency or dysfunction (e.g., from MTHFR mutations) can impair this process, causing systemic retention of histidine intermediates and reducing urinary histidine.
Thus in states of mitochondrial dysfunction (e.g., PDH impairment or TCA cycle disruption), it may be shunted toward pathways supporting mitochondrial function (e.g., ATP synthesis). It also may be linked to disruptions in nitrogen clearance pathways, as it can buffer excess nitrogen by contributing to the formation of ammonia or urea intermediates during metabolic stress and If aspartate is depleted it may be utilized as an alternative nitrogen donor to maintain nitrogen balance.
With elevated urinary glutamate this may signal a shift in amino acid metabolism that impacts histidine levels, so increased histidine catabolism to glutamate could deplete histidine, especially during mitochondrial or inflammatory stress. This pattern directly reflects glutamate dysfunction, with excess glutamate potentially leading to excitotoxicity.(57) Elevated glutamate levels have been associated with various neurological and psychiatric conditions, including autism spectrum disorders.(58)
These differing profiles likely represent distinct underlying mechanisms of glutamate dysfunction:
The first pattern may indicate issues with amino acid transport or metabolism, affecting related neurotransmitter systems without directly elevating glutamate.
The second pattern suggests a more direct problem with glutamate regulation, either through increased production, decreased clearance, or altered receptor function.(59)
The Urea Cycle
The urea cycle and the citric acid cycle are independent cycles but are linked. The urea cycle converts toxic nitrogenous compounds to excretable urea in five biochemical reactions. It is also the source for endogenous arginine, ornithine and citrulline production. The process mainly takes place in the liver, partly in the mitochondria and partly in the cytoplasm of the hepatocytes. The entire process converts two amino groups, one from NH+, and 1 from aspartate, and a carbon atom from HCO− to the relatively nontoxic excretion product urea. This occurs at the cost of four "high-energy"phosphate bonds (3 ATP hydrolyzed to 2 adenosine diphosphate (ADP) and one adenosine monophosphate (AMP). (83)
Figure 4: The Urea Cycle
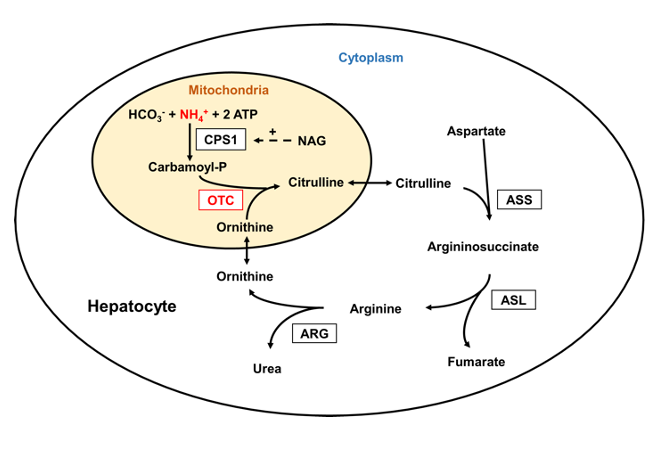
Source: Laemmle, Alexander; Gallagher, Renata C.; Keogh, Adrian; Stricker, Tamar; Gautschi, Matthias; Nuoffer, Jean-Marc; et al. (2016). Urea cycle.. PLOS ONE. Figure. https://doi.org/10.1371/journal.pone.0153358.g001 (84)
Amino Acid Dysfunction in catabolic “Burn Off”
Glutamate dysfunction has been identified in ME/CFS, Long COVID, Gulf War Syndrome, fibromyalgia, autism spectrum, ADHD, migraine, Alzheimer’s disease, Parkinson’s disease, and others. The neurotoxic changes from glutamate and other neuroexcitatory neurotransmitters identified in our amino acid studies appear to starting to answer many of the questions on the characteristic central sensitization that accompanies these conditions.
The convincing hypothesis of glutamate/astrocyte dysfunction by Guedl et al (40(41)) in Long COVID links neurotransmitter/amino acid neurotoxic changes with a variety of problems, notably Functional Neurological Disorder which is unfortunately thought of as a psychiatric problem, but reflects the damage caused by these neuroexcitatory/ neurotoxic amino acids, especially glutamate and aspartic acid following access through the BBB.
Examination of amino acid profiles using plasma and urinary assay demonstrates a number of response patterns. The neuroexcitatory amino acids glutamate and aspartic acid often complemented by low inhibitory glycine which may impact on increased neurotoxicity are frequently seen. The differences then provide a pathway to look at the metabolic differences that are occurring, and how these may be modulated. Excitatory amino acids like glutamate and aspartate can have neurotoxic effects when present in excessive amounts.
Trials of managing the known glutamate-associated conditions simply with low glutamate diets, especially in Gulf War Syndrome (GWS) veterans have proven to be largely ineffective, reflecting the complexity beyond the brief of the investigations. Most studies appeared to focus only on the glutamate pathways. By extending the investigations to include a broader range of amino acids (28) other neuroexcitatory dysfunction has been identified, eg glutamate and aspartic acid dysfunction implicated in autism spectrum disorder.(32)(35)
Our studies have also found dysfunction in aspartic acid, an excitatory amino acid neurotransmitter in the CNS, that stimulates NMDA receptors, though not as strongly as glutamate. Studies in 1960’s and 1970s in animal studies showed that oral intake of glutamate or aspartate could cause neuronal cell death in brain regions lacking a blood-brain-barrier (BBB).(42) This we believe becomes critical in the brain SPECT scans with the cerebral hyperperfusion reflecting endothelial dysfunction, disruption of the BBB and entry of the neuroexcitatory amino acid neurotransmitters into the brain seen in ME/CFS, POTS, and Long COVID.
The neurotoxic effects of neuroexcitatory amino acids like glutamate and aspartic acid appear to be mediated through excessive activation of glutamate receptors, especially NMDA receptors, leading to increased calcium influx and subsequent neuronal damage. Nitric oxide (NO) also appears to play a role.(42)
The NMDA (N-methyl-D-aspartate) receptor pathway, a glutamate and ion channel protein receptor that is activated when glycine and glutamate bind to it, is integral to excitatory neurotransmission in the brain, and its function can be influenced by various factors, including mutations in ion channels like TRP (transient receptor potential) channels. Mutations in TRP channels, as explored by Prof Marshall-Gradisnik and her team at National Centre for Neuroimmunology and Emerging Diseases (NCNED), Griffith University, can alter their function, potentially affecting calcium influx and homeostasis.
The team’s publications focussed primarily on the role of TRPM3 (Transient Receptor Potential Melastatin 3) ion channels in ME/CFS. They have demonstrated that TRPM3 channel activity is impaired in ME/CFS patients, particularly in Natural Killer (NK) cells. The dysfunction of TRPM3 channels affects calcium influx into cells, which is crucial for various cellular processes, including the function of NK cells. Their research has explored potential therapeutic interventions, including the use of low-dose naltrexone (LDN) to potentially improve TRPM3 function in ME/CFS patients.
There are potential approaches to mitigate aspartic acid neurotoxicity with dietary modification removing processed food containing aspartic acid, using antioxidants, phytochemicals such as curcumin and quercetin.(43)
The amino acid changes of catabolic dysfunction provide a potential pathway for management by metabolic profiling, particularly when the physical and other “drivers” in these conditions are identified and managed. The impact of diet and metabolic management is so very important as avoiding food the body sees as a threat reduces the cytokine response that drives the inflammation further, while simple treatments such as walking, improved posture and physical rehabilitation improves lymphatic flow and reduction of POTS symptoms.
There are differences from our amino acid findings and those found by Fluge et al (1), we believe as our focus has been on the range including POTS, Fibromyalgia Syndrome and Long COVID, where chronic fatigue is an active component, rather than just ME/CFS, which was their focus of the major research, so when combined with the DNA findings by Dr Vittone (3), dysfunctional metabolic pathways emerge.
Studies by Glass and Germain (68) investigating PEM, found differences in urinary metabolities in between controls and ME/CFS patients in many lipid (steroids, acyl carnitines and acyl glycines) and amino acid subpathways (cysteine, methionine, SAM, and taurine; leucine, isoleucine, and valine; polyamine; tryptophan; and urea cycle, arginine and proline). They discovered a lack of change in the urine metabolome of ME/CFS patients during recovery potentially demonstrating the lack of adaptation to a severe stress in ME/CFS patients. We anticipate this to reflect the hypoperfusion- induced catabolic metabolism found in Post Exertional Malaise (PEM).
Masoodi et al (69) found distinct alterations in lipid and amino acid metabolism in COVID patients, and identified sphingolipid, tryptophan, tyrosine, glutamine, arginine, and arachidonic acid metabolism as mostly impacted pathways. Notably, gamma-aminobutyric acid (GABA) was significantly reduced in COVID-19 patients, revealing large metabolic disturbances. (69)
Neuroexcitatory amino acids:
Adding GABA assays to the studies has shown a common pattern of low GABA, elevated plasma and urinary glutamate and low urinary aspartic acid, which suggests a bottleneck in the conversion of glutamate to GABA or utilization in the TCA cycle. This has wide reaching potential implications in cognitive impairment and functional neurological disorders that require more detailed research.
Under certain conditions excessive activation of excitatory amino acid receptors can lead to neurotoxicity, or “excitotoxicity.”(42) Excitotoxicity can occur from excessive release, overwhelming the normal mechanisms for clearance and reuptake, where the exacerbated or prolonged activation of glutamate receptors starts a cascade of neurotoxicity that ultimately leads to the loss of neuronal function and cell death. The molecular mechanism that triggers excitotoxicity involves alterations in glutamate and calcium metabolism, dysfunction of glutamate transporters, and malfunction of glutamate receptors, particularly N-methyl-D-aspartic acid receptors (NMDAR).
Impaired glutamate-glutamine cycle, when the astrocytes fail to effectively recycle glutamate leads to its accumulation in the extracellular space with neuroexcitatory symptoms. Excitotoxicity can be regarded as a consequence of other cellular phenomena, such as mitochondrial dysfunction, physical neuronal damage, and oxidative stress.(44)
Glutamate: Glutamate pathways comprise the major excitatory system in the brain and linked to many other neurotransmitter pathways, including GABA, the main inhibitory neurotransmitter. Glutamic acid (the anionic form is known as glutamate) is an α-amino acid that is used by almost all living beings in the biosynthesis of proteins. It is a non-essential nutrient for humans, meaning that the human body can synthesize enough for its use.-(discussed below) It is found in high concentrations throughout the brain and compartmentalized in synaptic vesicles for Ca2+-dependent release. It is the primary excitatory neurotransmitter in the brain, responsible for fast excitatory transmission, synaptic plasticity, learning and memory processes and sensory perception.(23) Under certain conditions excessive activation of excitatory amino acid receptors can lead to neurotoxicity, or “excitotoxicity.”(42) Excitotoxicity can occur:
Excessive release, overwhelming the normal mechanisms for clearance and reuptake, where the exacerbated or prolonged activation of glutamate receptors starts a cascade of neurotoxicity that ultimately leads to the loss of neuronal function and cell death. The molecular mechanism that triggers excitotoxicity involves alterations in glutamate and calcium metabolism, dysfunction of glutamate transporters, and malfunction of glutamate receptors, particularly N-methyl-D-aspartic acid receptors (NMDAR).
Impaired glutamate-glutamine cycle, when the astrocytes fail to effectively recycle glutamate leads to its accumulation in the extracellular space. On the other hand, excitotoxicity can be regarded as a consequence of other cellular phenomena, such as mitochondrial dysfunction, physical neuronal damage, and oxidative stress.(44)
Aspartic acid is an excitatory neurotransmitter and is involved in synthesis of other amino acids including methionine, threonine, isoleucine and lysine. It plays a role in the citric acid cycle for energy production and the urea cycle. It acts as an excitatory neurotransmitter in the ventral spinal cord similar to glycine (which is inhibitory).(37) Aspartate and glutamate can excite virtually every neuron in the CNS at low concentrations.(38) Aspartate is found in large quantities in neuroendocrine tissues eg hypothalamus. (39) Aspartate and glycine form an excitatory/inhibitory pain in the spinal cord, similar to glutamate/GABA in the brain.(37)
Histidine is essential in protein synthesis, tissue repair and production of red and white blood cells.
It is synthesized in the tissue by carnosine synthase from histidine and β-alanine, at the expense of ATP hydrolysis.
It plays an important role in enzymes eg serine proteases (such as trypsin.)
Histidine is required as a precursor of carnosine in human muscle and parts of the brain where carnosine appears to play an important role as a buffer and antioxidant. Carnosine is synthesized from histidine and β-alanine, at the expense of ATP hydrolysis. (45)
Carnosine plays an important role in exercise performance and skeletal muscle homeostasis. It is widely used among athletes in the form of supplements. It may play a significant role in PEM, but at present this is merely speculative. (46)
Histidine can be decarboxylated to histamine by histidine decarboxylase. This reaction occurs in the enterochromaffin-like cells of the stomach, in the mast cells of the immune system, and in various regions of the brain where histamine may serve as a neurotransmitter.
Inborn errors have been recognized in all of the catabolic enzymes of histidine. Histidine is required as a precursor of carnosine in human muscle and parts of the brain where carnosine appears to play an important role as a buffer and antioxidant. It can also be degraded to glutamate.(45)
Low levels of Pl histidine have been identified in PEM in our amino acid studies, which suggests, when Pl glutamate levels are elevated, increased catabolism of histidine to glutamate.
Histidine supplementation can be inappropriate in liver disease, with increases in ammonia and glutamine.
The increasing glutamate levels may increase the glutamate excitotoxicity.(347
Ethanolamine and Phospholipid Dysfunction
As data levels progress, the convoluted implications of the altered amino acids are becoming apparent, in particular in excitatory neurotransmission, and collagen formation and integrity.
Research has found a complex relationship between phospholipid dysfunction and COVID-19 severity. COVID-19 coagulopathy shares pathophysiologic features with antiphospholipid syndrome (APS), including endothelial dysfunction, platelet activation, complement activation, and neutrophil extracellular trap formation. Butt, Erkan and Lee (48) describe: “Antiphospholipid antibody production in COVID-19 is common, with 50% of COVID-19 patients being positive for lupus anticoagulant in some studies, and with non-Sapporo criteria antiphospholipid antibodies being prevalent as well. The biological significance of antiphospholipid antibodies in COVID-19 is uncertain, as such antibodies are usually transient, and studies examining clinical outcomes in COVID-19 patients with and without antiphospholipid antibodies have yielded conflicting results.”
Anti-phosphatidylserine/prothrombin (aPS/PT) IgG antibodies were significantly higher in COVID-19 patients with neurological symptoms compared to controls. (44) Ethanolamine plays an important role in phospholipid metabolism, and its dysfunction has been associated with phospholipid abnormalities observed in COVID-19 patients.
Ethanolamine is a component of cell membranes and involved in lipid metabolism. It is a precursor for phosphatidylethanolamine synthesis to phosphatidylcholine. Found very frequently in our amino acid studies, low urinary ethanolamine and high phosphoethanolamine levels suggest disruptions in phospholipid metabolism, potentially causing altered cell membrane composition, which could affect neuronal membrane stability and function, potentially impacting pain signalling, and ethanolamine is involved in choline synthesis, which is important for acetylcholine production, a neurotransmitter involved in pain modulation.(62)
PEMT mutations- Phosphatidylethanolamine N-methyltransferase (PEMT) catalyses phosphatidylcholine synthesis. PEMT and similar mutations are involved in vascular complications, neurodegeneration and thrombo-inflammation. It is thought that PEMT gene polymorphisms are associated with non-alcoholic fatty liver disease (NAFLD). Fatigue is a common symptom of PEMT mutations and its associated mitochondrial dysfunction, and is thought to be involved in neurodegenerative disease. PEMT is involved in the biosynthesis of phosphatidylcholine (PC) from phosphatidylethanolamine (PE), and likely to be the underlying culprit in persistent D-Dimer tests in Long Covid. A mutation in PEMT could potentially lead to an accumulation of phosphatidylethanolamine, and by extension affect ethanolamine levels. PEMT is a critical DNA mutation in POTS and Long COVID. (3)
The association between PEMT mutations and thromboinflammatory processes, especially as seen in COVID may explain some of the POTS and Long COVID symptoms. Ramya Dwivedi (49) describes that “vascular complications in COVID-patients links phosphatidylserine (PS) to thrombo-inflammation. Thrombo-inflammation plays a critical role through complement activation and cytokine release, platelet overactivity, apoptosis (thrombocytopathy), as well as coagulation abnormalities (coagulopathy).”(49)
Rauchet al (50) found phosphatidylserine was associated with increased thrombo-inflammation and vascular complications. The DNA mutations found by Dr Valerio Vittone (3) have contributed significantly to understanding the involved pathways, particularly in patients with DNA mutations eg PEMT There are no known biomarkers for this mutation, so abnormalities in this amino acid metabolism may provide a valuable link and a way to monitor metabolism and abnormalities in this pathway.
PEMT mutations could contribute to oxidative stress by altering membrane composition and function. This could affect cellular signalling, ion channel function and vascular integrity
Abnormalities in amino acid metabolism involving ethanolamine could be both a cause and a consequence of the metabolic disturbances associated with PEMT mutations
Relationship of fatty acid breakdown to phospholipid dysfunction in PEMT mutations
PEMT (phosphatidylethanolamine N-methyltransferase) mutations can significantly impact phospholipid metabolism and fatty acid distribution, leading to various physiological dysfunctions.
Phospholipid Metabolism Disruption
PEMT is a key enzyme responsible for converting phosphatidylethanolamine (PE) to phosphatidylcholine (PC) in the liver. Mutations in the PEMT gene can disrupt this conversion process, leading to:
Altered phospholipid composition: A decrease in PC and an accumulation of PE in cell membranes. In rats, a high-fat diet induces the selective distribution of fatty acids within phosphatidylcholine in the liver and muscles, in a manner that correlates with organ dysfunction. The cardiovascular system appears to be protected under these conditions (70)
Impaired membrane fluidity: Changes in the PC/PE ratio can affect membrane properties and function (70)
Fatty Acid Redistribution
The disruption in phospholipid metabolism caused by PEMT mutations can lead to abnormal fatty acid distribution:
Accumulation in specific phospholipids: Certain fatty acids, particularly polyunsaturated fatty acids (PUFAs), may accumulate in PE due to the reduced conversion to PC (70)
Altered fatty acid incorporation: The impaired PC synthesis can affect the incorporation of specific fatty acids into membrane phospholipids (71)
Metabolic Consequences
The interplay between fatty acid breakdown and phospholipid dysfunction in PEMT mutations can result in various metabolic issues:
Lipid droplet formation: Excess fatty acids may be stored in lipid droplets, potentially leading to hepatic steatosis (70)(72)
Mitochondrial dysfunction: Altered phospholipid composition can impair mitochondrial function, affecting fatty acid oxidation (72)
Insulin sensitivity: Changes in membrane phospholipid composition may impact insulin signalling and glucose metabolism (73)
Physiological Impacts
The disruption of phospholipid metabolism and fatty acid distribution can have broader physiological effects:
Liver dysfunction: PEMT mutations are associated with an increased risk of fatty liver disease and other hepatic disorders (70)
Cardiovascular risk: Alterations in plasma phospholipid fatty acid profiles may influence cardiovascular disease risk (74)
Phospholipid imbalance and cardiovascular risk.
The disruption of phospholipid metabolism, particularly the ratio of phosphatidylcholine (PC) to phosphatidylethanolamine (PE), can impact cardiovascular health. An altered PC/PE ratio can affect membrane properties and function, potentially leading to increased cardiovascular disease risk. (76)
Fatty acid metabolism and membrane composition:
Alterations in fatty acid metabolism, particularly in the synthesis and incorporation of polyunsaturated fatty acids (PUFAs) into membrane phospholipids, can affect cardiovascular health. Changes in the fatty acid composition of phosphatidylethanolamine in cell membranes have been observed in animal models of metabolic disorder (77)
3. Neurological effects: Phospholipid imbalances can potentially impact brain function, as phospholipids play crucial roles in neurotransmission and membrane integrity
In conclusion, PEMT mutations can lead to a complex interplay between fatty acid metabolism and phospholipid dysfunction. This relationship affects various cellular processes, potentially contributing to metabolic disorders, liver dysfunction, and other physiological impairments. Understanding these interactions is crucial for developing targeted therapies and interventions for individuals with PEMT mutations.
Amino Acids as described by Fluge et al (1)
Fluge (1) Category II:
Amino acids that enter the oxidation pathway as acetyl-CoA, which directly and independently of PDH fuels the TCA cycle for degradation to CO2.
Isoleucine (Ile) One of the three BCAAs, isoleucine is involved in muscle metabolism and is heavily concentrated in muscle tissue. It’s also important for immune function, haemoglobin production, and energy regulation.
Leucine (Leu) is a BCAA that is critical for protein synthesis and muscle repair. It also helps regulate blood sugar levels, stimulates wound healing, and produces growth hormones.(32)
Lysine (Lys) is vital for protein synthesis, hormone production and immune function. Low lysine levels may be caused by prolonged stress, too much arginine or histidine supplementation competing for absorption, or carnitine deficiency, as described above.
Phenylalanine (Phe) Your body turns this amino acid into the neurotransmitters tyrosine, dopamine, epinephrine, and norepinephrine. It plays an integral role in the structure and function of proteins and enzymes and the production of other amino acids.(32)
Tryptophan (Trp) Often associated with drowsiness, tryptophan is a precursor to serotonin, a neurotransmitter that regulates your appetite, sleep, and mood.(32) (see below)
Tyrosine (Tyr) is a precursor for neurotransmitters dopamine, noradrenalin and adrenalin. It is involved in melanin production, helps in synthesis of thyroid hormones, and supports functioning of the adrenal, thyroid and pituitary glands.(32) Tyrosine dysfunction particularly due to tyrosine hydroxylase, affects the conversion of tyrosine to L-Dopa, the direct precursor of dopamine. Dysfunction in tyrosine hydroxylase can result in neurological symptoms eg dystonia, tremor and Parkinson’s disease.
Fluge (1) Category I:
Amino acids are converted to pyruvate, and therefore depend on PDH to be further oxidized.
Alanine (Ala)- clears toxins released during muscle protein breakdown, aids in regulating blood glucose and cholesterol, serves as an energy source for muscles and the CNS and assist in lymphocyte production
Cysteine (Cys)- stimulates collagen production and is involved in the synthesis of glutathione, and plays a role in protein folding and stability
Glycine (Gly)- is involved in the production of DNA, phospholipids and collagen. It helps in the production of bile acids and supports glutathione synthesis. It acts as an inhibitory neurotransmitter in the CNS. Aspartate and glycine form an excitatory/inhibitory pain in the spinal cord, similar to glutamate/GABA in the brain.(20)
Serine (Ser)- is involved in phospholipid formation, is a precursor of several amino acids including glycine and cysteine. It plays a role in DNA and RNA synthesis, and is important for proper functioning of the CNS.
Threonine (Thr)- is a principal part of structural proteins such as collagen and elastin, which are important components of your skin and connective tissue. It also plays a role in fat metabolism and immune function.
The amino acids needed for ATP production and utilization include: (54)
Glutamine which can be converted into glucose through gluconeogenesis, then used to produce ATP
Alanine serves as a substrate for gluconeogenesis, especially when fasting or intense exercise
Arginine plays a role in the urea cycle and can be converted into nitric oxide that is involved in various signalling pathways influencing energy metabolism, as well as contributing to the synthesis of creatine which helps in ATL production
Methionine is involved in the synthesis of S-adenosylmethionine (SAMe), a critical donor in biochemical reactions, including those affecting ATP production and energy metabolism. DNA mutations in this have consistently been seen by DNA studies by Dr Valerio Vittone.(3)
Leucine activates the mTOR pathway that promotes protein synthesis and cellular growth, indirectly supporting ATP production by enhancing muscle recovery and energy utilization
Cysteine is important for synthesizing glutathione, an anti-oxidant that protects cells from oxidative stress which can impair ATP synthesis
Glycine is involved in the synthesis of creatine, which is crucial for ATP regeneration in muscle cells.
Other amino acids potentially involving the thromboinflammatory pathway:
Arginine, a precursor for nitric oxide, which plays a role in vascular function and inflammation
Glutamine, with its role in immune function and inflammatory responses
Cysteine important for glutathione synthesis, which is crucial for antioxidant defence
Tryptophan involved in kynurenine pathway -linked to inflammation and oxidative stress (see below)
Glycine has anti-inflammatory properties and is involved in haeme synthesis
Methionine is important for methylation reactions and can affect homocysteine levels, linked to cardiovascular risk
Targeting the “Perfect Storm”
The interplay between amino acid depletion, mitochondrial dysfunction, post exertional malaise (PEM) hypermetabolism, and brainstem hypoperfusion creates a “perfect storm.” As we go have progressed through the DNA findings, and adding the findings from the amino acid studies, we can look towards targeted metabolic solutions when required.
The effect of catabolic metabolism from PEM, generally aligned with brainstem hypoperfusion:
Muscle Protein Breakdown: PEM triggers muscle catabolism, releasing amino acids that are rapidly utilized, leading to systemic depletion.
Nitrogen Overload and Urea Cycle Stress, where increased utilization of aspartate depletes its systemic levels, impairing nitrogen clearance and contributing to metabolic stress.
Gut Barrier Dysfunction where appropriate: Increased intestinal permeability worsens systemic inflammation and impairs amino acid absorption.
Mitochondrial Dysfunction where reduced energy production forces reliance on amino acids for energy, further depleting their stores.
Neuroexcitation and BBB Breakdown: Elevated glutamate and impaired aspartate in the CNS, coupled with BBB dysfunction, drive excitotoxicity and localized hypermetabolism in the brain.
The combination of low GABA, low aspartic acid, elevated glutamate, ethanolamine, and lysine, alongside abnormal histidine, which I describe as the “Perfect Storm,” reflects a convergence of increased urea cycle utilization, muscle protein breakdown, mitochondrial dysfunction, and systemic inflammation. Similar amino acid abnormalities can be seen in a range of metabolic, inflammatory, and neurological conditions.
Mitochondrial Dysfunction with impairments in the TCA cycle and amino acid metabolism.
Neurotransmitter Imbalance, with excess excitatory signalling (glutamate) and reduced inhibitory signalling (GABA).
Metabolic Stress with dysregulation in nitrogen balance (aspartate, glutamate) and membrane metabolism (ethanolamine).
Ethanolamine is a component of cell membranes and involved in lipid metabolism. It is a precursor for phosphatidylethanolamine synthesis to phosphatidylcholine. Found very frequently in our amino acid studies, low urinary ethanolamine and high phosphoethanolamine levels suggest disruptions in phospholipid metabolism, potentially causing altered cell membrane composition, which could affect neuronal membrane stability and function, potentially impacting pain signalling.
Ethanolamine is involved in choline synthesis, which is important for acetylcholine production, a neurotransmitter involved in pain modulation. This dysfunction exacerbates the metabolic stress, neuroinflammation, and barrier breakdown observed in conditions like PEM, CFS, and brain hypoperfusion, and is associated with phospholipid dysfunction.
Lysine in preliminary studies is the most commonly effected amino acid. Lysine intake is crucial for maintaining healthy collagen function, which supports the structural integrity of skin, tendons, bones, and other connective tissues. Deficiencies can lead to weakened connective tissues, delayed wound healing, and other related health issues.
Sirtuin 4 (SIRT4) is a mitochondrial protein that inhibits mitochondrial glutamate dehydrogenase 1(GDH) activity, thereby down-regulating insulin secretion in response to amino acids, as well as PDH activity. This regulation prevents overactivation of PDHC under nutrient surplus conditions, preserving mitochondrial homeostasis.
Nicotinamide, as a precursor for NAD⁺, directly influences SIRT4 activity and mitochondrial metabolism. The interplay between nicotinamide, NAD⁺, and SIRT4 can influence the observed amino acid imbalances. Nicotinamide, by restoring NAD⁺ levels and modulating SIRT4, could break this "perfect storm" by enhancing mitochondrial efficiency, normalizing amino acid metabolism, and balancing neurotransmitter levels. Nicotinamide is available in a number of forms, eg normal Vitamin B3, nicotinamide, has been used for skin cancer modulation for many years.
Other amino acids may be abnormal, such as proline. Proline is a major component of collagen, and high plasma levels may signal increased collagen turnover or connective tissue remodeling. High proline also contributes to glutamate accumulation, exacerbating excitotoxicity and limiting downstream utilization in the TCA cycle.
Restoring the NAD+ levels can enhance the proline oxidation pathway, helping to regulate the proline-glutamate interconversion. NAD+ also supports the malate-aspartate shuttle, helping to address aspartate depletion. NAD+ supports urea cycle efficiency, helping clear ammonia that may accumulate from amino acid imbalances (e.g., proline and lysine dysregulation).
Clinic studies have found introducing liposomal nicotinamide riboside has positive effects on fatigue and amino acid patterns, including stabilizing of ethanolamine function. Liposomal delivery systems significantly enhance the bioavailability and clinical efficacy of supplements, including nicotinamide riboside (NR) for mitochondrial and metabolic dysfunction (as seen in CFS) and vitamin B3 (nicotinamide/niacin) for skin health and cancer prevention.
Therapeutic Potential of Nicotinamide and SIRT4 Modulation
Restores NAD⁺ Levels by boosting NAD⁺ which supports mitochondrial metabolism, improving TCA cycle function, amino acid metabolism, and neurotransmitter balance.
Inhibits SIRT4 (Indirectly) at high concentrations, relieving GDH inhibition and enhancing glutamate clearance.
Supports Neurotransmitter Balance as improved glutamate metabolism increases GABA synthesis, addressing excitotoxicity and anxiety.
Stabilizes Amino Acid Metabolism as replenished mitochondrial function improves aspartate production, lysine metabolism, and ethanolamine utilization.
Reduces Mast Cell Activation by reducing inflammatory signalling, stabilizing histidine/histamine balance.
GABA and low Aspartic acid
In managing CFS patients with abnormal amino acid profiles, the addition of nicotinamide riboside has shown promise in restoring levels of lysine, ethanolamine, and valine to normal ranges. However, the persistently low urinary aspartic acid levels, believed to be reflective of underlying hypoxia during post-exertional malaise (PEM), remain a challenge. This imbalance with glutamate can lead to neuroexcitation, and while lowering dietary glutamate intake can be beneficial, additional pathways and management strategies may be helpful.
To address low aspartic acid levels, Dunstan et al (91) proposed supplementation with N-acetylcysteine (NAC) may help increase aspartate levels by promoting the conversion of cysteine to cystine, which can then be used to synthesize aspartate. (92) The potential benefits of NAC for ME/CFS patients, particularly in addressing oxidative stress and potentially improving symptoms, are promising. However, the risks, while generally mild, should not be overlooked. (98)
Supporting the urea cycle with supplements like arginine or citrulline may help increase aspartate production, although the important research by Weigel et al (92) found that the role of nutritional intake and supplement use on ME/CFS patients’ health-related quality of life remains unclear, dietary changes and the use of supplements appear to be of value to ME/CFS patients.
With the addition of GABA to the amino acid assays, low levels of GABA are being consistently seen. There is evidence supports the idea that increasing GABA levels can help counteract the excitatory effects of glutamate and potentially reduce neuroexcitation. There may be insufficient conversion of glutamate to GABA due to impaired glutamate decarboxylase (GAD) activity or pyridoxal-5’-phosphate (active B6) deficiency. If B6 levels are low (and care must be taken to ensure avoiding neurotoxicity from excess B6, Pyridoxal-5’-phosphate (P5P) starting at 25 to 50 mg daily may be helpful.
Additionally, there is evidence for the role of magnesium in glutamate regulation. Magnesium plays a significant role in regulating glutamate activity, as magnesium acts as a natural NMDA receptor antagonist, which can help regulate glutamate activity, and by blocking the NMDA receptor channel, magnesium can reduce excessive glutamate signalling.
Magnesium's action at NMDA receptors can protect against glutamate-induced excitotoxicity, which is implicated in various neurological disorders. (93) Magnesium can influence the release of neurotransmitters, including glutamate, potentially helping to maintain proper excitatory/inhibitory balance.(96) The impact of magnesium supplementation may vary depending on an individual's baseline magnesium levels and overall neurological health. Suggested is magnesium glycinate form, 200 to 400mg daily.
While the evidence supports the potential benefits of increasing GABA levels (and magnesium supplementation) in regulating glutamate activity, the relationship between these neurotransmitters is complex and context-dependent. While increasing GABA levels and magnesium supplementation show promise in regulating glutamate excitation, their effects can be nuanced and dependent on various factors. For example, in some cases, GABA can be excitatory, particularly during early development or in certain pathological conditions. (97) The effectiveness of GABA in counteracting glutamate excitation can depend on factors such as intracellular chloride concentrations and the expression of specific transporters. (94)(97)
CoQ10 at 100 to 300 mg daily has been shown may support mitochondrial function alongside nicotinic acid, the combination more effective than each alone in neuroprotection, blocking ATP deletions and lactate increases.(105) Yet nicotinamide and NAD+, nor randomly applied supplementation, while may improve fatigue, will not solve the metabolic problems. Further improvements can be made when targeting relevant genetic mutations—including those affecting mitochondrial functions, inflammatory pathways such as MCAS, nuclear factor kappa beta, interleukin 6, TNF alpha, and interleukin 1 beta, derived from the DNA investigations by Dr Valerio Vittone.
While detailed family history can provide some genetic risk information, individualized DNA through Dr Vittone provides far more information into the various gene mutations than the normal commercially available products, and have been providing valuable insights into these complex pathways. These can provide a springboard into nutraceutical treatments.
Targeted diagnostics and personalized therapeutic approaches, which include dietary and nutraceutical protocols combined with mitochondrial and mast cell support, offers a promising strategy for addressing these complex biochemical disturbances. The targeted approach may help address the underlying metabolic imbalances and mitigate their clinical consequences. For the future, there is a peptide product currently highly regulated in Australia, which if used appropriately, in combination with a targeted management protocol, could yield significant improvements in many areas, thus warranting formal research.
Linking Amino Acid Depletion, Metabolic Dysfunction, PEM, Brainstem hypoperfusion and Cerebral hypermetabolism (77)
The Tryptophan-Kynurenine Metabolic Pathway (sourced from Szabó A, Tanaka, M, Torok, N, Vecsei,A. The Tryptophan-Kynurenine Pathway. Scholarly Community Encyclopedia (64))
Tryptophan (TRP) is one of the essential amino acids, which participates in protein synthesis. The tryptophan’s main metabolic route is the kynurenine pathway (KP) through which approximately 90-95% of TRP degrades into nicotinamide adenine dinucleotide (NAD+) and other bioactive metabolites. The appropriate amount of NAD+ is essential to maintain the operation and the viability of cells. The other molecules are neurotoxic, neuroprotective, oxidant, antioxidant, and/or immune modifiers. They play an important role in the function of the brain and the peripheral tissues. The change in the levels of the bioactive molecules is considered to contribute to the development of a wide range of illnesses from cancer to immunologic, neurodegenerative, and psychiatric diseases.
TRP is a precursor to essential biomolecules such as NAD+, serotonin (5-HT), and melatonin (MT), among others. 5-HT and MT are synthesized through the methoxyindole pathway. However, only 1-5% of TRP is degraded in the route. Nearly 95% of TRP is utilized for the KP, leading to the synthesis of NAD+ and other bioactive molecules. NAD+ is a cofactor of the electron transport in the respiratory chain, which is essential for the synthesis of adenosine triphosphate (ATP). NAD+ also plays a remarkable role in several enzyme reactions and in the brain’s glycogen storage.
In addition to NAD+, several bioactive molecules are synthesized in the KP, contributing to appropriate functions in the brain as well in the body. In the past years, researchers have been revealing that these molecules play physiologically important roles in the functions of life and observing disproportional change in the levels of the molecules in illness. Thus, it is considered that KP metabolites play crucial roles in pathogenesis and disease progression.
Figure 5: The Tryptophan-kynurenine metabolic pathway and the metabolites

Source: Török N, Tanaka M, Vécsei L. Searching for Peripheral Biomarkers in Neurodegenerative Diseases: The Tryptophan-Kynurenine Metabolic Pathway. (63)
Previous studies showed that alterations in TRP metabolism were associated with tumours and immunologic, neurodegenerative, and psychiatric diseases. The enzymes of the KP were observed up-regulated in tumour cells of the brain, breast, and pancreas. Patients suffering from cutaneous malignant melanoma showed significantly lower levels of 3-HK and 3-HAA but a higher level of KYN. Colon cancer cells showed an increased level of KYN but decreased levels of the other metabolites. In immunologic diseases rheumatoid arthritis and systemic lupus erythematosus (SLE) the increases in some KP intermediates.
Altered levels of KP metabolites have been also observed in patients with neurodegenerative diseases such as Alzheimer’s disease, Huntington’s disease (HD), Parkinson’s disease, multiple sclerosis, amyotrophic lateral sclerosis, human immunodeficiency virus (HIV)-associated neurocognitive disorders, stroke-induced secondary neurodegeneration, and vascular cognitive dementia. Furthermore, alterations of the KP have been reported in psychiatric diseases including depressive disorder, bipolar disorder, anxiety disorder, schizophrenia (SCZ), aggressive behaviour, and autism spectrum disorder.
Figure 6: Kynurenine levels in tumours, immunologic diseases, neurodegenerative diseases, and psychiatric disorders
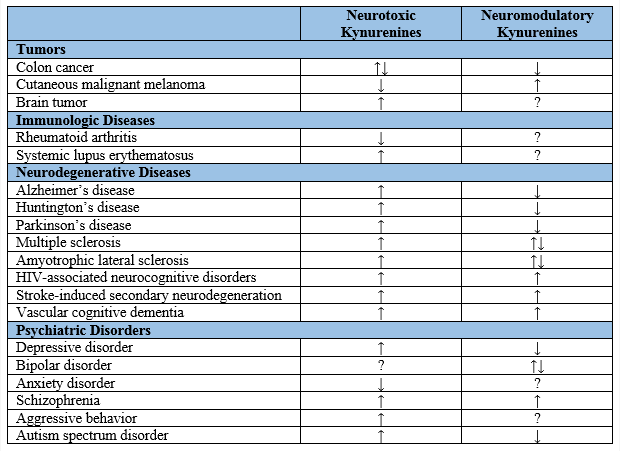
Source: Szabó A, Tanaka, M, Torok, N, Vecsei,A. The Tryptophan-Kynurenine Pathway. Scholarly Community Encyclopedia (64)
PDH Dysfunction, Lactate and ADHD
The emergence of the importance of pyruvate dehydrogenase dysfunction in CFS by Fluge et al also has particular relevance in ADHD, as data emerges of the doubling of ADHD from COVID.(85) A meta-analysis of 18 studies from 10 countries found that many children and/or their caregivers reported an increase in child ADHD symptoms during the COVID-19 pandemic. Studies have shown exacerbated problems with depressive behaviours, school avoidance, and difficulties with online learning among youth with ADHD during the pandemic. COVID-19 stress has been associated with increased ADHD symptom severity, particularly hyperactivity/impulsivity symptoms, in longitudinal studies. (86)
Australia has experienced a substantial rise in ADHD diagnoses and medication use, with ADHD medication prescriptions more than doubling in the past five years, from 1.4 million prescriptions for 186,000 people in 2018 to 3.2 million prescriptions for 414,000 people in 2022. Factors contributing to this rise include increased awareness through social media, celebrity diagnoses, and the COVID-19 pandemic. (87)
The amino acid studies have shown the dysfunctional Fluge Category 1 amino acids early studies are showing elevated pyruvate levels that are metabolized to lactate, a primary factor in ASD and ADHD. Research into the “Lactate Shuttle” by Brookes et al (89) may be reflected in a different way to assess and treat ASD and ADHD.
The ”Lactate Shuttle” facilitates exchange of lactate between astrocytes and neurons in the brain. Within cells lactate exchanged between cytosol and mitochondria, and between cytosol and peroxisomes which has a role in phospholipid synthesis for nerve cell myelination. NAD+ may improve “Lactate Shuttle” function.
The complex linking between these is discussed in ADHD- another link between POTS and Chronic Fatigue Syndrome.(88)
Associated Vitamin Deficiencies
When amino acids are low, a number of vitamins may be low or in increased demand.
Vitamin B6 (pyridoxine) is important for neurotransmitter synthesis and immune function may be depleted by increased metabolic demands. It is a required cofactor for amino acid metabolism.
L dopa and tryptophan are dietary derived. Amino acid decarboxylase is also B6 dependant.
Symptoms of B6 deficiency:
Skin rashes, especially seborrhoeic dermatitis
Cracked sore lips and angle of mouth
Anaemia and fatigue
Depression and confusion
Immune dysfunction
Neurological symptoms eg neuropathic pain and numbness
Symptoms of B6 toxicity: this is almost never caused by dietary intake, but usually by high-dose supplements.
Peripheral neuropathy
Painful skin lesions
Photosensitivity
Nausea
Impaired body movements
Vitamin B12 (cobalamin) works closely with folate in amino acid metabolism and protein synthesis. The impact of PEM on B12 is not fully understood but potential impacts include:
Increased oxidative stress with breakdown of amino acids, indirectly affecting B12 metabolism and methylation reactions
Mitochondrial dysfunction. PEM involves mitochondrial dysfunction and B12 is crucial for cellular energy production, this dysfunction interfering with B12 utilization at a cellular level
Altered methylation. B12 is essential for methylation processes, and the metabolic stress in PEM may increase the demand for methylation reactions, affecting B12 utilization and availability
Impaired B12 absorption with the association with gut dysfunction in CFS
· Altered energy metabolism and potential for B12 deficiency
Folate (vitamin B9) essential for metabolism of several amino acids including histidine, glycine and serine
Vitamin C (ascorbic acid) Vitamin C, a powerful antioxidant may be affected by PEM as the body attempts to deal with the increased oxidative stress, and a higher utilization.
The “burn off” or catabolism of amino acids in PEM could indirectly affect Vitamin C dependent pathways, eg metabolism of Tyrosine.
Collagen synthesis may be affected by the PEM’s demand for vitamin C
The immune stress in PEM may increase the demand for Vitamin C to maintain immune defences
Vitamin B3 (niacin) is involved in the synthesis of tryptophan. Low niacin can affect tryptophan breakdown and serotonin production.
Vitamin B1 (thiamine) plays a role in metabolism of branched chain acids leucine, isoleucine and valine.
Vitamin D which is important for calcium absorption, immune function and neuromuscular health may be affected by altered absorption and increased utilization
Vitamin E an antioxidant protecting cell membranes may be depleted in oxidative stress
Associated Mineral Deficiencies
Zinc is essential for immune function, wound healing, DNA synthesis and is a cofactor for numerous enzymes. In a “burn off” potential mechanisms include increased demand from chronic inflammation, altered absorption or increased excretion and utilization in antioxidant processes.
Magnesium is critical for energy production, nerve function and muscle contraction. With depletion from increased metabolic demands
Selenium an antioxidant supporting immune function nand thyroid hormone metabolism may be depleted by increased oxidative stress
Copper is necessary for iron metabolism and antioxidant function, and may be altered by altered absorption and increased utilization
Manganese plays an important role in collagen formation and function and is required for the activation of prolidase an enzyme crucial for collagen synthesis
Major Neurotransmitter Pathways
The balance and interplay between these different neurotransmitter pathways are crucial for normal brain function, and disruptions can lead to neurological and psychiatric disorders.
Dopamine pathways- involved in reward, motivation and motor control. Dopamine is the primary neurotransmitter in the mesolimbic (reward) pathway, involved in motivation, pleasure and reinforcement of behaviours. Dopamine pathways are critical for motor function, with disruptions leading to Parkinson’s Disease.
Serotonin pathways- projections to various parts of the nervous system influencing functions such as sleep, memory, appetite and mood. It is involved in regulating sleep-wake cycles.
Glutamate pathways- the major excitatory system in the brain and linked to many other neurotransmitter pathways, including GABA, the main inhibitory neurotransmitter. Glutamate is involved in numerous pathways related to learning, memory and cognitive function.(18)
GABA (Gamma-aminobutyric acid) is the main inhibitory neurotransmitter in the CNS, helping to regulate and balance neural activity. GABA pathways are involved in managing anxiety and stress responses.
Acetylcholine (Ach) pathways are crucial for maintaining cognitive function, with disruptions linked to Alzheimer’s disease. In the peripheral nervous system Ach is essential for muscle activation.
Noradrenaline pathways are involved in regulating arousal, attention and fight-or flight responses. It plays a key role in the sympathetic nervous system influencing heart rate, blood pressure and other autonomic functions
Histamine pathways are involved in regulating the sleep-wake cycle and promoting wakefulness. Histamine pathways are also involved in immune responses and brain inflammation.
Conclusion
We provide a preliminary assessment of ongoing amino acid assays where brainstem hypoperfusion, coat hanger pain and PEM is present, that same group of medical conditions linked by fatigue and cognitive impairment- ME/CFS, POTS, Fibromyalgia Syndrome, Gulf War Syndrome and Long COVID. Research into coat hanger pain has provided pathways demonstrating where hypoperfusion induces muscle depolarization, weakness, and mitochondrial dysfunction.
ME/CFS patients have reduced reserves of Adenosine triphosphate (ATP) vital for mitochondrial energy production, and replenishment of ATP may take days. In post exertional malaise (PEM) the metabolic dysfunction induced by the hypoperfusion moves the body into catabolic metabolism with increased “burn off” of amino acids, essential minerals and vitamins.
The hypoxia is believed to impair the function of enzyme pyruvate dehydrogenase (PDH) in ME/CFS causing changes in the vital Krebs/ Citric acid cycle responsible for our mitochondrial energy source leading to inadequate adenosine triphosphate (ATP) generation by oxidative phosphorylation and excessive lactate generation on exertion.(1)
Glutamate metabolism is complex and interacts with many other biochemical pathways. The specific amino acid profiles found in these studies may provide clues about the nature of the dysfunction, potentially guiding treatment approaches.(42) For example, addressing amino acid imbalances or targeting glutamate receptors may be more appropriate depending on the observed pattern. These patterns highlight the heterogeneity of glutamate-related disorders and the need for personalized approaches in diagnosis and treatment. Further research is needed to fully understand the implications of these amino acid profiles and their relationship to specific symptoms or conditions.
Persistent low urinary aspartic acid with varying levels of glutamate suggestingdysfunction in the body’s use and replenishment of aspartate, possibly with dysfunction in the Malate Aspartate Shuttle may explain the characteristic delays in timing of PEM. Targeting the Perfect Storm. (90)
I believe these preliminary studies will foster a more detailed research that affects ME/CFS, POTS, Fibromyalgia as well as Long COVID and lay the path for improved management protocols.
References:
1. Fluge Ø, Mella O, Bruland O, Risa K, Dyrstad SE, Alme K, Rekeland IG, Sapkota D, Røsland GV, Fosså A, Ktoridou-Valen I, Lunde S, Sørland K, Lien K, Herder I, Thürmer H, Gotaas ME, Baranowska KA, Bohnen LM, Schäfer C, McCann A, Sommerfelt K, Helgeland L, Ueland PM, Dahl O, Tronstad KJ. Metabolic profiling indicates impaired pyruvate dehydrogenase function in myalgic encephalopathy/chronic fatigue syndrome. JCI Insight. 2016 Dec 22;1(21):e89376. doi: 10.1172/jci.insight.89376. PMID: 28018972; PMCID: PMC5161229.
2. Hoel F, Hoel A, Pettersen IK, Rekeland IG, Risa K, Alme K, Sørland K, Fosså A, Lien K, Herder I, Thürmer HL, Gotaas ME, Schäfer C, Berge RK, Sommerfelt K, Marti HP, Dahl O, Mella O, Fluge Ø, Tronstad KJ. A map of metabolic phenotypes in patients with myalgic encephalomyelitis/chronic fatigue syndrome. JCI Insight. 2021 Aug 23;6(16):e149217. doi: 10.1172/jci.insight.149217. PMID: 34423789; PMCID: PMC8409979.
3. Vittone, V., Exelby, G. DNA Mutations the Underpin POTS and Long COVID. 2024. https://www.mcmc-research.com/post/dna-mutations-that-underpin-pots-and-long-covid
4. Johnson,C. “Straining for Energy”- Large Metabolic Study Suggests ME/CFS is an “immunometabolic” Disease. Health Rising. 2021. https://www.healthrising.org/blog/2021/12/08/energy-chronic-fatigue-syndrome-immunometabolic-disease/
5. Brand MD, Orr AL, Perevoshchikova IV, Quinlan CL. The role of mitochondrial function and cellular bioenergetics in ageing and disease. Br J Dermatol. 2013 Jul;169 Suppl 2(0 2):1-8. doi: 10.1111/bjd.12208. PMID: 23786614; PMCID: PMC4321783.
6. Wang X, Shen X, Yan Y, Li H. Pyruvate dehydrogenase kinases (PDKs): an overview toward clinical applications. Biosci Rep. 2021 Apr 30;41(4):BSR20204402. doi: 10.1042/BSR20204402. PMID: 33739396; PMCID: PMC8026821.
7. Jeon JH, Thoudam T, Choi EJ, Kim MJ, Harris RA, Lee IK. Loss of metabolic flexibility as a result of overexpression of pyruvate dehydrogenase kinases in muscle, liver and the immune system: Therapeutic targets in metabolic diseases. J Diabetes Investig. 2021 Jan;12(1):21-31. doi: 10.1111/jdi.13345. Epub 2020 Sep 10. PMID: 32628351; PMCID: PMC7779278.
8. Wirth, K.J., Scheibenbogen, C. & Paul, F. An attempt to explain the neurological symptoms of Myalgic Encephalomyelitis/Chronic Fatigue Syndrome. J Transl Med 19, 471 (2021). https://doi.org/10.1186/s12967-021-03143-3
9. Thapaliya K, Marshall-Gradisnik S, Barth M, Eaton-Fitch N, Barnden L. Brainstem volume changes in myalgic encephalomyelitis/chronic fatigue syndrome and long COVID patients. Front Neurosci. 2023 Mar 2;17:1125208. doi: 10.3389/fnins.2023.1125208. PMID: 36937672; PMCID: PMC10017877.
10. Thapaliya K, Marshall-Gradisnik S, Eaton-Fitch N, Eftekhari Z, Inderyas M, Barnden L. Imbalanced Brain Neurochemicals in Long COVID and ME/CFS: A Preliminary Study Using MRI. Am J Med. 2024 Apr 6:S0002-9343(24)00216-X. doi: 10.1016/j.amjmed.2024.04.007. Epub ahead of print. PMID: 38588934.
11. Verger, A., Kas, A., Dudouet, P. et al. Visual interpretation of brain hypometabolism related to neurological long COVID: a French multicentric experience. Eur J Nucl Med Mol Imaging 49, 3197–3202 (2022). https://doi.org/10.1007/s00259-022-05753-5
12. Hulens M, Dankaerts W, Rasschaert R, Bruyninckx F, De Mulder P, Bervoets C. The Link Between Empty Sella Syndrome, Fibromyalgia, and Chronic Fatigue Syndrome: The Role of Increased Cerebrospinal Fluid Pressure. J Pain Res. 2023;16:205-219https://doi.org/10.2147/JPR.S394321
13. Bragée B, Michos A, Drum B, Fahlgren M, Szulkin R, Bertilson BC. Signs of Intracranial Hypertension, Hypermobility, and Craniocervical Obstructions in Patients With Myalgic Encephalomyelitis/Chronic Fatigue Syndrome. Front Neurol. 2020 Aug 28;11:828. doi: 10.3389/fneur.2020.00828. PMID: 32982905; PMCID: PMC7485557.
14. Exelby,G. Brainstem Hypoperfusion, Coat Hanger pain and Post-Exertional Malaise in POTS and Long COVID. 2024. https://www.mcmc-research.com/post/brainstem-hypoperfusion-coat-hanger-pain-and-post-exertional-malaise-in-pots-and-long-covid
15. Appelman, B., Charlton, B.T., Goulding, R.P. et al. Muscle abnormalities worsen after post-exertional malaise in long COVID. Nat Commun 15, 17 (2024). https://doi.org/10.1038/s41467-023-44432-3
16. Humm AM, Bostock H, Troller R, Z'Graggen WJ. Muscle ischaemia in patients with orthostatic hypotension assessed by velocity recovery cycles. J Neurol Neurosurg Psychiatry. 2011 Dec;82(12):1394-8. doi: 10.1136/jnnp-2011-300444. Epub 2011 Jun 7. PMID: 21653205.
17. McGregor NR, Armstrong CW, Lewis DP, Gooley PR. Post-Exertional Malaise Is Associated with Hypermetabolism, Hypoacetylation and Purine Metabolism Deregulation in ME/CFS Cases. Diagnostics (Basel). 2019 Jul 4;9(3):70. doi: 10.3390/diagnostics9030070. PMID: 31277442; PMCID: PMC6787670.
18. Germain A, Giloteaux L, Moore GE, Levine SM, Chia JK, Keller BA, Stevens J, Franconi CJ, Mao X, Shungu DC, Grimson A, Hanson MR. Plasma metabolomics reveals disrupted response and recovery following maximal exercise in myalgic encephalomyelitis/chronic fatigue syndrome. JCI Insight. 2022 May 9;7(9):e157621. doi: 10.1172/jci.insight.157621. PMID: 35358096; PMCID: PMC9090259.
19. Glass KA, Germain A, Huang YV, Hanson MR. Urine Metabolomics Exposes Anomalous Recovery after Maximal Exertion in Female ME/CFS Patients. Int J Mol Sci. 2023 Feb 12;24(4):3685. doi: 10.3390/ijms24043685. PMID: 36835097; PMCID: PMC9958671.
20. Exelby, G. Causes of Long COVID Cognitive Impairment. (2024) https://www.mcmc-research.com/post/causes-of-long-covid-cognitive-impairment
21. van Eeden C, Khan L, Osman MS, Cohen Tervaert JW. Natural Killer Cell Dysfunction and Its Role in COVID-19. Int J Mol Sci. 2020 Sep 1;21(17):6351. doi: 10.3390/ijms21176351. PMID: 32883007; PMCID: PMC7503862.
22. Ndwandwe C, Schwarze J, Shannon E, Sokolowska M, Sadlier C, O'Mahony L. Immune Mechanisms Underpinning Long COVID: Collegium Internationale Allergologicum Update 2024. Int Arch Allergy Immunol. 2024;185(5):489-502. doi: 10.1159/000535736. Epub 2024 Jan 22. PMID: 38253027.
23. Exelby, G. Intracranial Hypertension, Intracranial Hypotension, CSF Leaks and Craniovascular Pressure Change. 2024. https://www.mcmc-research.com/post/intracranial-hypertension-intracranial-hypotension-and-craniovascular-pressure-change
24. Song J, da Costa KA, Fischer LM, et al. Polymorphism of the PEMT gene and susceptibility to nonalcoholic fatty liver disease (NAFLD). FASEB J. 2005;19(10):1266-1271. doi:10.1096/fj.04-3580com
25.Malengier-Devlies B, Filtjens J, Ahmadzadeh K, Boeckx B, Vandenhaute J, De Visscher A, Bernaerts E, Mitera T, Jacobs C, Vanderbeke L, Van Mol P, Van Herck Y, Hermans G, Meersseman P, Wilmer A, Gouwy M, Garg AD, Humblet-Baron S, De Smet F, Martinod K, Wauters E, Proost P, Wouters C, Leclercq G, Lambrechts D, Wauters J, Matthys P. Severe COVID-19 patients display hyper-activated NK cells and NK cell-platelet aggregates. Front Immunol. 2022 Oct 5;13:861251. doi: 10.3389/fimmu.2022.861251. PMID: 36275702; PMCID: PMC9581751.
26.Di Vito C, Calcaterra F, Coianiz N, Terzoli S, Voza A, Mikulak J, Della Bella S, Mavilio D. Natural Killer Cells in SARS-CoV-2 Infection: Pathophysiology and Therapeutic Implications. Front Immunol. 2022 Jun 30;13:888248. doi: 10.3389/fimmu.2022.888248. PMID: 35844604; PMCID: PMC9279859.
27. Chandel NS. Amino Acid Metabolism. Cold Spring Harb Perspect Biol. 2021 Apr 1;13(4):a040584. doi: 10.1101/cshperspect.a040584. PMID: 33795250; PMCID: PMC8015690.
28. Debevec-McKenney, Marshall, T. Amino Acid Metabolism. Osmosis. https://www.osmosis.org/learn/Amino_acid_metabolism
29. Stansley BJ, Yamamoto BK. L-Dopa and Brain Serotonin System Dysfunction. Toxics. 2015 Mar 5;3(1):75-88. doi: 10.3390/toxics3010075. PMID: 29056652; PMCID: PMC5634697
30. Silkaitis RP, Mosnaim AD. Pathways linking L-phenylalanine and 2-phenylethylamine with p-tyramine in rabbit brain. Brain Res. 1976 Sep 10;114(1):105-15. doi: 10.1016/0006-8993(76)91010-6. PMID: 963535.
31. Tomen, D. Phenethylamine (PEA). Nootropicsexpert. 2023. https://nootropicsexpert.com/phenylethylamine/
32. Kubala,J. Essential Amino Acids: Definition, Benefits, and Food Sources. Healthline. 2023. https://www.healthline.com/nutrition/essential-amino-acids
33. Teloh, J.K., Dohle, DS., Petersen, M. et al. Histidine and other amino acids in blood and urine after administration of Bretschneider solution (HTK) for cardioplegic arrest in patients: effects on N-metabolism. Amino Acids 48, 1423–1432 (2016). https://doi.org/10.1007/s00726-016-2195-2
34. Jukić I, Kolobarić N, Stupin A, Matić A, Kozina N, Mihaljević Z, Mihalj M, Šušnjara P, Stupin M, Ćurić ŽB, Selthofer-Relatić K, Kibel A, Lukinac A, Kolar L, Kralik G, Kralik Z, Széchenyi A, Jozanović M, Galović O, Medvidović-Kosanović M, Drenjančević I. Carnosine, Small but Mighty-Prospect of Use as Functional Ingredient for Functional Food Formulation. Antioxidants (Basel). 2021 Jun 28;10(7):1037. doi: 10.3390/antiox10071037. PMID: 34203479; PMCID: PMC8300828.
35. Lopez MJ, Mohiuddin SS. Biochemistry, Essential Amino Acids. [Updated 2024 Apr 30]. In: StatPearls [Internet]. Treasure Island (FL): StatPearls Publishing; 2024 Jan-. Available from: https://www.ncbi.nlm.nih.gov/books/NBK557845/
36. The Essentials of Nonessential Amino Acids. Aminoco. 2018. https://aminoco.com/blogs/amino-acids/essentials-nonessential-amino-acids
37. Patri M (2019) Synaptic Transmission and Amino Acid Neurotransmitters. Neurochemical Basis of Brain Function and Dysfunction. IntechOpen. http://dx.doi.org/10.5772/intechopen.82121.
38. Dingledine R, McBain CJ. Glutamate and Aspartate Are the Major Excitatory Transmitters in the Brain. In: Siegel GJ, Agranoff BW, Albers RW, et al., editors. Basic Neurochemistry: Molecular, Cellular and Medical Aspects. 6th edition. Philadelphia: Lippincott-Raven; 1999. : https://www.ncbi.nlm.nih.gov/books/NBK28252/
39. van den Pol AN, Wuarin JP, Dudek FE. Glutamate, the dominant excitatory transmitter in neuroendocrine regulation. Science. 1990 Nov 30;250(4985):1276-8. doi: 10.1126/science.1978759. PMID: 1978759.
40. Verger, A., Kas, A., Dudouet, P. et al. Visual interpretation of brain hypometabolism related to neurological long COVID: a French multicentric experience. Eur J Nucl Med Mol Imaging 49, 3197–3202 (2022). https://doi.org/10.1007/s00259-022-05753-5
41. Hotowitz,T, Pellurin,L, Zimmer, E, Guedj,E. Brain fog in long COVID: A glutamatergic hypothesis with astrocyte dysfunction accounting for brain PET glucose hypometabolism. Elsevier, Medical Hypotheses. https://doi.org/10.1016/j.mehy.2023.111186
42. Gillessen T, Budd SL, Lipton SA. Excitatory Amino Acid Neurotoxicity. In: Madame Curie Bioscience Database [Internet]. Austin (TX): Landes Bioscience; 2000-2013. Available from: https://www.ncbi.nlm.nih.gov/books/NBK6108/
43. Rajeh, N.A. Mechanistic progression of acrylamide neurotoxicity linked to neurodegeneration and mitigation strategies. Discov Appl Sci 6, 181 (2024). https://doi.org/10.1007/s42452-024-05850-0
44. Armada-Moreira A, Gomes JI, Pina CC, Savchak OK, Gonçalves-Ribeiro J, Rei N, Pinto S, Morais TP, Martins RS, Ribeiro FF, Sebastião AM, Crunelli V, Vaz SH. Going the Extra (Synaptic) Mile: Excitotoxicity as the Road Toward Neurodegenerative Diseases. Front Cell Neurosci. 2020 Apr 24;14:90. doi: 10.3389/fncel.2020.00090. PMID: 32390802; PMCID: PMC7194075.
45. Brosnan ME, Brosnan JT. Histidine Metabolism and Function. J Nutr. 2020 Oct 1;150(Suppl 1):2570S-2575S. doi: 10.1093/jn/nxaa079. PMID: 33000155; PMCID: PMC7527268.
46. Jukić I, Kolobarić N, Stupin A, Matić A, Kozina N, Mihaljević Z, Mihalj M, Šušnjara P, Stupin M, Ćurić ŽB, Selthofer-Relatić K, Kibel A, Lukinac A, Kolar L, Kralik G, Kralik Z, Széchenyi A, Jozanović M, Galović O, Medvidović-Kosanović M, Drenjančević I. Carnosine, Small but Mighty-Prospect of Use as Functional Ingredient for Functional Food Formulation. Antioxidants (Basel). 2021 Jun 28;10(7):1037. doi: 10.3390/antiox10071037. PMID: 34203479; PMCID: PMC8300828.
47. Melis GC, ter Wengel N, Boelens PG, van Leeuwen PA. Glutamine: recent developments in research on the clinical significance of glutamine. Curr Opin Clin Nutr Metab Care. 2004 Jan;7(1):59-70. doi: 10.1097/00075197-200401000-00011. PMID: 15090905.
48. Butt A, Erkan D, Lee AI. COVID-19 and antiphospholipid antibodies. Best Pract Res Clin Haematol. 2022 Sep;35(3):101402. doi: 10.1016/j.beha.2022.101402. Epub 2022 Oct 15. PMID: 36494152; PMCID: PMC9568270.
49. Dwivedi,R. Researchers study COVID-19 immune dysfunction in relation to phosphatidylserine. https://www.news-medical.net/news/20210623/Researchers-study-COVID-19-immune-dysfunction-in-relation-to-phosphatidylserine.aspx
50. Rauch, L et al. Binding of phosphatidylserine-positive microparticles by PBMCs classifies disease severity in COVID-19 patients. 2021 bioRxiv. doi: https://doi.org/10.1101/2021.06.18.448935
51. Yamauchi M, Sricholpech M. Lysine post-translational modifications of collagen. Essays Biochem. 2012;52:113-33. doi: 10.1042/bse0520113. PMID: 22708567; PMCID: PMC3499978.
52. Shoulders MD, Raines RT. Collagen structure and stability. Annu Rev Biochem. 2009;78:929-58. doi: 10.1146/annurev.biochem.77.032207.120833. PMID: 19344236; PMCID: PMC2846778.
53. Guzyk MM, Sergiichuk IuT, Dyakun KO, Yanitska LV, Kuchmerovska TM. Effect of nicotinamide on amino acids content in bone collagen depending on biological availability of vitamins in diabetic rats. Ukr Biochem J. 2014 Jul-Aug;86(4):138-49. doi: 10.15407/ubj86.04.138. PMID: 25509193.
54. El Bacha, T., Luz, M. & Da Poian, A. (2010) Dynamic Adaptation of Nutrient Utilization in Humans. Nature Education 3. https://www.nature.com/scitable/topicpage/dynamic-adaptation-of-nutrient-utilization-in-humans-14232807/
55. Rumping L, Vringer E, Houwen RHJ, van Hasselt PM, Jans JJM, Verhoeven-Duif NM. Inborn errors of enzymes in glutamate metabolism. J Inherit Metab Dis. 2020 Mar;43(2):200-215. doi: 10.1002/jimd.12180. Epub 2019 Oct 11. PMID: 31603991; PMCID: PMC7078983.
56. Chen, WX., Chen, YR., Peng, MZ. et al. Plasma Amino Acid Profile in Children with Autism Spectrum Disorder in Southern China: Analysis of 110 Cases. J Autism Dev Disord 54, 1567–1581 (2024). https://doi.org/10.1007/s10803-022-05829-z
57. Jiawen Wang MD, Yingmei Zhang MD, Ning Tian MD, Dongshan Ya MD, Jiaxin Yang MD, Yanlin Jiang MD, Xiaoxia Li MD, Xiaohui Lin MD, Bin Yang PhD, Qinghua Li PhD, Rujia Liao PhD. Mechanism of glutamate metabolic function and dysfunction in vascular dementia. Neuroptrotection, Wiley Online Library, 2024. https://doi.org/10.1002/nep3.32
58. Ahmad Ghanizadeh. Increased Glutamate and Homocysteine and Decreased Glutamine Levels in Autism: A Review and Strategies for Future Studies of Amino Acids in Autism. Wiley Online Library, 2013. https://doi.org/10.1155/2013/536521
59. Andersen JV, Markussen KH, Jakobsen E, Schousboe A, Waagepetersen HS, Rosenberg PA, Aldana BI. Glutamate metabolism and recycling at the excitatory synapse in health and neurodegeneration. Neuropharmacology. 2021 Sep 15;196:108719. doi: 10.1016/j.neuropharm.2021.108719. Epub 2021 Jul 15. PMID: 34273389.
60. Kavyani, B., Lidbury, B.A., Schloeffel, R. et al. Could the kynurenine pathway be the key missing piece of Myalgic Encephalomyelitis/Chronic Fatigue Syndrome (ME/CFS) complex puzzle?. Cell. Mol. Life Sci. 79, 412 (2022). https://doi.org/10.1007/s00018-022-04380-5
61. Naviaux RK, Naviaux JC, Li K, Bright AT, Alaynick WA, Wang L, Baxter A, Nathan N, Anderson W, Gordon E. Metabolic features of chronic fatigue syndrome. Proc Natl Acad Sci U S A. 2016 Sep 13;113(37):E5472-80. doi: 10.1073/pnas.1607571113. Epub 2016 Aug 29. Erratum in: Proc Natl Acad Sci U S A. 2017 May 2;114(18):E3749. doi: 10.1073/pnas.1703858114. PMID: 27573827; PMCID: PMC5027464.
63. Löhn, M., Wirth, K.J. Potential pathophysiological role of the ion channel TRPM3 in myalgic encephalomyelitis/chronic fatigue syndrome (ME/CFS) and the therapeutic effect of low-dose naltrexone. J Transl Med 22, 630 (2024). https://doi.org/10.1186/s12967-024-05412-3
64.Szabó A, Tanaka, M, Torok, N, Vecsei,A. The Tryptophan-Kynurenine Pathway. Scholarly Community Encyclopedia. 2021. https://encyclopedia.pub/entry/7774
65Germain A, Ruppert D, Levine SM, Hanson MR. Metabolic profiling of a myalgic encephalomyelitis/chronic fatigue syndrome discovery cohort reveals disturbances in fatty acid and lipid metabolism. Mol Biosyst. 2017 Jan 31;13(2):371-379. doi: 10.1039/c6mb00600k. PMID: 28059425; PMCID: PMC5365380.
66.De Luca, A., Pierno, S. & Camerino, D.C. Taurine: the appeal of a safe amino acid for skeletal muscle disorders. J Transl Med 13, 243 (2015). https://doi.org/10.1186/s12967-015-0610-1
67. Nadeem Rais, Akash Ved, Mohd. Shadab, Rizwan Ahmad, Mohammad Shahid. Taurine, a non-proteinous essential amino acid for human body systems: an overview. Arab Gulf Journal of Scientific Research. 2022. https://www.emerald.com/insight/content/doi/10.1108/AGJSR-04-2022-0019/full/html
68. Glass KA, Germain A, Huang YV, Hanson MR. Urine Metabolomics Exposes Anomalous Recovery after Maximal Exertion in Female ME/CFS Patients. Int J Mol Sci. 2023 Feb 12;24(4):3685. doi: 10.3390/ijms24043685. PMID: 36835097; PMCID: PMC9958671.
69. Masoodi M, Peschka M, Schmiedel S, Haddad M, Frye M, Maas C, Lohse A, Huber S, Kirchhof P, Nofer JR, Renné T. Disturbed lipid and amino acid metabolisms in COVID-19 patients. J Mol Med (Berl). 2022 Apr;100(4):555-568. doi: 10.1007/s00109-022-02177-4. Epub 2022 Jan 22. PMID: 35064792; PMCID: PMC8783191.
70. Bacle A, Kadri L, Khoury S, Ferru-Clément R, Faivre JF, Cognard C, Bescond J, Krzesiak A, Contzler H, Delpech N, Colas J, Vandebrouck C, Sébille S, Ferreira T. A comprehensive study of phospholipid fatty acid rearrangements in metabolic syndrome: correlations with organ dysfunction. Dis Model Mech. 2020 Jun 15;13(6):dmm043927. doi: 10.1242/dmm.043927. PMID: 32303571; PMCID: PMC7328154.
71. Danielli M, Perne L, Jarc Jovičić E, Petan T. Lipid droplets and polyunsaturated fatty acid trafficking: Balancing life and death. Front Cell Dev Biol. 2023 Jan 27;11:1104725. doi: 10.3389/fcell.2023.1104725. Erratum in: Front Cell Dev Biol. 2023 Mar 22;11:1175493. doi: 10.3389/fcell.2023.1175493. PMID: 36776554; PMCID: PMC9911892.
72. Hwang, H.M., Kawasawa, Y.I., Basha, A. et al. Fatty acid metabolism changes in association with neurobehavioral deficits in animal models of fetal alcohol spectrum disorders. Commun Biol 6, 736 (2023). https://doi.org/10.1038/s42003-023-05127-z
73. Borkman M, Storlien LH, Pan DA, Jenkins AB, Chisholm DJ, Campbell LV. The relation between insulin sensitivity and the fatty-acid composition of skeletal-muscle phospholipids. N Engl J Med. 1993 Jan 28;328(4):238-44. doi: 10.1056/NEJM199301283280404. PMID: 8418404.
74. Matthan NR, Ooi EM, Van Horn L, Neuhouser ML, Woodman R, Lichtenstein AH. Plasma phospholipid fatty acid biomarkers of dietary fat quality and endogenous metabolism predict coronary heart disease risk: a nested case-control study within the Women's Health Initiative observational study. J Am Heart Assoc. 2014 Aug 13;3(4):e000764. doi: 10.1161/JAHA.113.000764. PMID: 25122663; PMCID: PMC4310362.
75. Horrobin DF, Bennett CN. Depression and bipolar disorder: relationships to impaired fatty acid and phospholipid metabolism and to diabetes, cardiovascular disease, immunological abnormalities, cancer, ageing and osteoporosis. Possible candidate genes. Prostaglandins Leukot Essent Fatty Acids. 1999 Apr;60(4):217-34. doi: 10.1054/plef.1999.0037. PMID: 10397403.
76. Li J, Xin Y, Li J, Chen H, Li H. Phosphatidylethanolamine N-methyltransferase: from Functions to Diseases. Aging Dis. 2023 Jun 1;14(3):879-891. doi: 10.14336/AD.2022.1025. PMID: 37191416; PMCID: PMC10187709.
77. Hwang, H.M., Kawasawa, Y.I., Basha, A. et al. Fatty acid metabolism changes in association with neurobehavioral deficits in animal models of fetal alcohol spectrum disorders. Commun Biol 6, 736 (2023). https://doi.org/10.1038/s42003-023-05127-z
Yamano E, Sugimoto M, Hirayama A, Kume S, Yamato M, Jin G, Tajima S, Goda N, Iwai K, Fukuda S, Yamaguti K, Kuratsune H, Soga T, Watanabe Y, Kataoka Y. Index markers of chronic fatigue syndrome with dysfunction of TCA and urea cycles. Sci Rep. 2016 Oct 11;6:34990. doi: 10.1038/srep34990. PMID: 27725700; PMCID: PMC5057083.
79. Chambers,P. Long COVID, POTS, CFS, and MTHFR: Lifting the Fog? Journal of Neurology & Neurophysiology. 2023. DOI:10.35248/2332-2594.23.14(3).341
80. Germain A, Ruppert D, Levine SM, Hanson MR. Metabolic profiling of a myalgic encephalomyelitis/chronic fatigue syndrome discovery cohort reveals disturbances in fatty acid and lipid metabolism. Mol Biosyst. 2017 Jan 31;13(2):371-379. doi: 10.1039/c6mb00600k. PMID: 28059425; PMCID: PMC5365380.
81. Yamauchi M, Sricholpech M. Lysine post-translational modifications of collagen. Essays Biochem. 2012;52:113-33. doi: 10.1042/bse0520113. PMID: 22708567; PMCID: PMC3499978.
82. Tomé D, Moro J, Blais A, Chaumontet C, Even P, Piedcoq J, Gaudichon C, Delhaye N, Azzout-Marniche D. Impact of Low Protein and Lysine-deficient Diets on Bone Metabolism (P08-072-19). Curr Dev Nutr. 2019 Oct 24;3(Suppl 1):nzz044.P08-072-19. doi: 10.1093/cdn/nzz044.P08-072-19. PMCID: PMC6818847.
Urea Cycle. Wikipedia. https://en.wikipedia.org/wiki/Urea_cycle
Laemmle, Alexander; Gallagher, Renata C.; Keogh, Adrian; Stricker, Tamar; Gautschi, Matthias; Nuoffer, Jean-Marc; et al. (2016). Urea cycle.. PLOS ONE. Figure. https://doi.org/10.1371/journal.pone.0153358.g001
Auro K, Holopainen I, Perola M, Havulinna AS, Raevuori A. Attention-Deficit/Hyperactivity Disorder Diagnoses in Finland During the COVID-19 Pandemic. JAMA Netw Open. 2024 Jun 3;7(6):e2418204. doi: 10.1001/jamanetworkopen.2024.18204. PMID: 38935377; PMCID: PMC11211961.
Rogers MA, MacLean J. ADHD Symptoms Increased During the Covid-19 Pandemic: A Meta-Analysis. J Atten Disord. 2023 Jun;27(8):800-811. doi: 10.1177/10870547231158750. Epub 2023 Mar 6. PMID: 36879524; PMCID: PMC9996113.
The Age. Why has everyone suddenly got ADHD? Australian Psychological Society. 2024. https://psychology.org.au/about-us/news-and-media/aps-in-the-media/2024/why-has-everyone-suddenly-got-adhd
Exelby, G. ADHD- another link between POTS and Chronic Fatigue Syndrome. 2024. https://www.mcmc-research.com/post/adhd-another-link-between-pots-and-chronic-fatigue-syndrome
Brooks GA, Curl CC, Leija RG, Osmond AD, Duong JJ, Arevalo JA. Tracing the lactate shuttle to the mitochondrial reticulum. Exp Mol Med. 2022 Sep;54(9):1332-1347. doi: 10.1038/s12276-022-00802-3. Epub 2022 Sep 8. PMID: 36075947; PMCID: PMC9534995.
Exelby,G. Linking Amino Acid Depletion, Metabolic Dysfunction, PEM, brainstem hypoperfusion and cerebral hypermetabolism.2024. https://www.mcmc-research.com/post/linking-amino-acid-depletion-metabolic-dysfunction-pem-brainstem-hypoperfusion-and-cerebral-hyper
Dunstan RH, Sparkes DL, Roberts TK, Crompton MJ, Gottfries J, Dascombe BJ. Development of a complex amino acid supplement, Fatigue Reviva™, for oral ingestion: initial evaluations of product concept and impact on symptoms of sub-health in a group of males. Nutr J. 2013;12:115. Published 2013 Aug 8. doi:10.1186/1475-2891-12-115
Weigel B, Eaton-Fitch N, Passmore R, Cabanas H, Staines D, Marshall-Gradisnik S. A preliminary investigation of nutritional intake and supplement use in Australians with myalgic encephalomyelitis/chronic fatigue syndrome and the implications on health-related quality of life. Food Nutr Res. 2021;65:10.29219/fnr.v65.5730. Published 2021 Jun 7. doi:10.29219/fnr.v65.5730
Gamma-aminobutyric acid (GABA). Cleveland Clinic. https://my.clevelandclinic.org/health/articles/22857-gamma-aminobutyric-acid-gaba
de Leon AS, Tadi P. Biochemistry, Gamma Aminobutyric Acid. [Updated 2023 May 1]. In: StatPearls [Internet]. Treasure Island (FL): StatPearls Publishing; 2024 Jan-. Available from: https://www.ncbi.nlm.nih.gov/books/NBK551683/
van den Pol AN, Gao XB, Patrylo PR, Ghosh PK, Obrietan K. Glutamate inhibits GABA excitatory activity in developing neurons. J Neurosci. 1998;18(24):10749-10761. doi:10.1523/JNEUROSCI.18-24-10749.1998
Sears SM, Hewett SJ. Influence of glutamate and GABA transport on brain excitatory/inhibitory balance. Exp Biol Med (Maywood). 2021;246(9):1069-1083. doi:10.1177/1535370221989263
Kilb W. When Are Depolarizing GABAergic Responses Excitatory?. Front Mol Neurosci. 2021;14:747835. Published 2021 Nov 24. doi:10.3389/fnmol.2021.747835
Assessment of N-Acetylcysteine as Therapy for Myalgic Encephalomyelitis/Chronic Fatigue Syndrome. National Institute of Neurological Disorders and Stroke. https://www.ninds.nih.gov/health-information/clinical-trials/assessment-n-acetylcysteine-therapy-myalgic-encephalomyelitischronic-fatigue-syndrome
Lu M, Zhou L, Stanley WC, Cabrera ME, Saidel GM, Yu X. Role of the malate-aspartate shuttle on the metabolic response to myocardial ischemia. J Theor Biol. 2008;254(2):466-475. doi:10.1016/j.jtbi.2008.05.033
Xu S, Zhang X, Liu C, Liu Q, Chai H, Luo Y, Li S. Role of Mitochondria in Neurodegenerative Diseases: From an Epigenetic Perspective. Front Cell Dev Biol. 2021 Aug 27;9:688789. doi: 10.3389/fcell.2021.688789. PMID: 34513831; PMCID: PMC8429841.
Molnar T, Lehoczki A, Fekete M, et al. Mitochondrial dysfunction in long COVID: mechanisms, consequences, and potential therapeutic approaches. Geroscience. 2024;46(5):5267-5286. doi:10.1007/s11357-024-01165-5
Broeks MH, van Karnebeek CDM, Wanders RJA, Jans JJM, Verhoeven-Duif NM. Inborn disorders of the malate aspartate shuttle. J Inherit Metab Dis. 2021;44(4):792-808. doi:10.1002/jimd.12402
Leng A, Shah M, Ahmad SA, et al. Pathogenesis Underlying Neurological Manifestations of Long COVID Syndrome and Potential Therapeutics. Cells. 2023;12(5):816. Published 2023 Mar 6. doi:10.3390/cells12050816
Weisshaar, N., Ma, S., Ming, Y. et al. The malate shuttle detoxifies ammonia in exhausted T cells by producing 2-ketoglutarate. Nat Immunol 24, 1921–1932 (2023). https://doi.org/10.1038/s41590-023-01636-5
Beal MF, Henshaw DR, Jenkins BG, Rosen BR, Schulz JB. Coenzyme Q10 and nicotinamide block striatal lesions produced by the mitochondrial toxin malonate. Ann Neurol. 1994 Dec;36(6):882-8. doi: 10.1002/ana.410360613. PMID: 7998775.
Kommentare